This is a preprint.
Mapping and engineering RNA-controlled architecture of the multiphase nucleolus
- PMID: 39386460
- PMCID: PMC11463421
- DOI: 10.1101/2024.09.28.615444
Mapping and engineering RNA-controlled architecture of the multiphase nucleolus
Abstract
Biomolecular condensates are key features of intracellular compartmentalization. As the most prominent nuclear condensate in eukaryotes, the nucleolus is a layered multiphase liquid-like structure and the site of ribosome biogenesis. In the nucleolus, ribosomal RNAs (rRNAs) are transcribed and processed, undergoing multiple maturation steps that ultimately result in formation of the ribosomal small subunit (SSU) and large subunit (LSU). However, how rRNA processing is coupled to the layered nucleolar organization is poorly understood due to a lack of tools to precisely monitor and perturb nucleolar rRNA processing dynamics. Here, we developed two complementary approaches to spatiotemporally map rRNA processing and engineer de novo nucleoli. Using sequencing in parallel with imaging, we found that rRNA processing steps are spatially segregated, with sequential maturation of rRNA required for its outward movement through nucleolar phases. Furthermore, by generating synthetic de novo nucleoli through an engineered rDNA plasmid system in cells, we show that defects in SSU processing can alter the ordering of nucleolar phases, resulting in inside-out nucleoli and preventing rRNA outflux, while LSU precursors are necessary to build the outermost layer of the nucleolus. These findings demonstrate how rRNA is both a scaffold and substrate for the nucleolus, with rRNA acting as a programmable blueprint for the multiphase architecture that facilitates assembly of an essential molecular machine.
Conflict of interest statement
Competing interests C.P.B. is a scientific founder, Scientific Advisory Board member, shareholder, and consultant for Nereid Therapeutics.
Figures
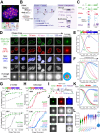
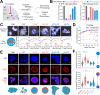
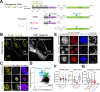
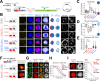
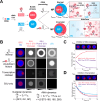
Similar articles
-
Viscoelasticity and advective flow of RNA underlies nucleolar form and function.Mol Cell. 2023 Sep 7;83(17):3095-3107.e9. doi: 10.1016/j.molcel.2023.08.006. Mol Cell. 2023. PMID: 37683610 Free PMC article.
-
The nucleolus as a polarized coaxial cable in which the rDNA axis is surrounded by dynamic subunit-specific phases.Curr Biol. 2021 Jun 21;31(12):2507-2519.e4. doi: 10.1016/j.cub.2021.03.041. Epub 2021 Apr 15. Curr Biol. 2021. PMID: 33862007 Free PMC article.
-
The nucleolar protein NOL12 is required for processing of large ribosomal subunit rRNA precursors in Arabidopsis.BMC Plant Biol. 2023 Nov 3;23(1):538. doi: 10.1186/s12870-023-04561-9. BMC Plant Biol. 2023. PMID: 37919659 Free PMC article.
-
The nucleolus.Anat Embryol (Berl). 1993 Dec;188(6):515-36. doi: 10.1007/BF00187008. Anat Embryol (Berl). 1993. PMID: 8129175 Review.
-
The functional organization of the nucleolus in proliferating plant cells.Eur J Histochem. 2000;44(2):117-31. Eur J Histochem. 2000. PMID: 10968360 Review.
References
-
- Shin Y. & Brangwynne C. P. Liquid phase condensation in cell physiology and disease. Science 357, (2017). - PubMed
-
- Brangwynne C. P., Tompa P. & Pappu R. V. Polymer physics of intracellular phase transitions. Nat. Phys. 11, 899–904 (2015).
-
- Pappu R. V., Cohen S. R., Dar F., Farag M. & Kar M. Phase Transitions of Associative Biomacromolecules. Chem. Rev. 123, 8945–8987 (2023). - PubMed
Publication types
Grants and funding
LinkOut - more resources
Full Text Sources
Research Materials