A fluid-walled microfluidic platform for human neuron microcircuits and directed axotomy
- PMID: 38841815
- PMCID: PMC11198392
- DOI: 10.1039/d4lc00107a
A fluid-walled microfluidic platform for human neuron microcircuits and directed axotomy
Abstract
In our brains, different neurons make appropriate connections; however, there remain few in vitro models of such circuits. We use an open microfluidic approach to build and study neuronal circuits in vitro in ways that fit easily into existing bio-medical workflows. Dumbbell-shaped circuits are built in minutes in standard Petri dishes; the aqueous phase is confined by fluid walls - interfaces between cell-growth medium and an immiscible fluorocarbon, FC40. Conditions are established that ensure post-mitotic neurons derived from human induced pluripotent stem cells (iPSCs) plated in one chamber of a dumbbell remain where deposited. After seeding cortical neurons on one side, axons grow through the connecting conduit to ramify amongst striatal neurons on the other - an arrangement mimicking unidirectional cortico-striatal connectivity. We also develop a moderate-throughput non-contact axotomy assay. Cortical axons in conduits are severed by a media jet; then, brain-derived neurotrophic factor and striatal neurons in distal chambers promote axon regeneration. As additional conduits and chambers are easily added, this opens up the possibility of mimicking complex neuronal networks, and screening drugs for their effects on connectivity.
Conflict of interest statement
P. R. C. and E. J. W. co-founded, and hold equity in, IotaSciences Ltd. The same company provides financial support to F. N., plus reagents and equipment for the study. Oxford University Innovation – the technology transfer company of The University of Oxford – has filed patent applications on behalf of P. R. C. and E. J. W. covering technologies used in this study.
Figures
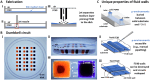

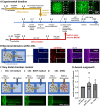
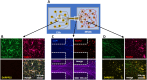
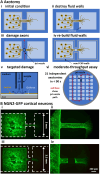
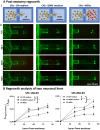
Similar articles
-
Live Imaging Analysis of Axonal Regeneration in Human iPSC-Derived Motor Neurons Using a Microfluidic System.Methods Mol Biol. 2024;2831:333-350. doi: 10.1007/978-1-0716-3969-6_23. Methods Mol Biol. 2024. PMID: 39134861
-
Microfluidics on Standard Petri Dishes for Bioscientists.Small Methods. 2021 Nov;5(11):e2100724. doi: 10.1002/smtd.202100724. Epub 2021 Oct 7. Small Methods. 2021. PMID: 34927960 Review.
-
A microfluidic platform to study the effects of GDNF on neuronal axon entrapment.J Neurosci Methods. 2018 Oct 1;308:183-191. doi: 10.1016/j.jneumeth.2018.08.002. Epub 2018 Aug 3. J Neurosci Methods. 2018. PMID: 30081039 Free PMC article.
-
Axon diodes for the reconstruction of oriented neuronal networks in microfluidic chambers.Lab Chip. 2011 Nov 7;11(21):3663-73. doi: 10.1039/c1lc20014c. Epub 2011 Sep 15. Lab Chip. 2011. PMID: 21922081
-
The role of neurotrophic factors in nerve regeneration.Neurosurg Focus. 2009 Feb;26(2):E3. doi: 10.3171/FOC.2009.26.2.E3. Neurosurg Focus. 2009. PMID: 19228105 Review.
Cited by
-
Stable diffusion gradients in microfluidic conduits bounded by fluid walls.Microsyst Nanoeng. 2024 Jun 20;10:79. doi: 10.1038/s41378-024-00698-1. eCollection 2024. Microsyst Nanoeng. 2024. PMID: 38911344 Free PMC article.
-
The dynamics of capillary flow in an open-channel system featuring trigger valves.bioRxiv [Preprint]. 2024 Nov 30:2024.09.17.613325. doi: 10.1101/2024.09.17.613325. bioRxiv. 2024. Update in: Sci Rep. 2024 Dec 30;14(1):31732. doi: 10.1038/s41598-024-82329-3 PMID: 39345588 Free PMC article. Updated. Preprint.
References
Publication types
MeSH terms
Substances
LinkOut - more resources
Full Text Sources
Research Materials