Neutron diffraction from a microgravity-grown crystal reveals the active site hydrogens of the internal aldimine form of tryptophan synthase
- PMID: 38645802
- PMCID: PMC11027755
- DOI: 10.1016/j.xcrp.2024.101827
Neutron diffraction from a microgravity-grown crystal reveals the active site hydrogens of the internal aldimine form of tryptophan synthase
Abstract
Pyridoxal 5'-phosphate (PLP), the biologically active form of vitamin B6, is an essential cofactor in many biosynthetic pathways. The emergence of PLP-dependent enzymes as drug targets and biocatalysts, such as tryptophan synthase (TS), has underlined the demand to understand PLP-dependent catalysis and reaction specificity. The ability of neutron diffraction to resolve the positions of hydrogen atoms makes it an ideal technique to understand how the electrostatic environment and selective protonation of PLP regulates PLP-dependent activities. Facilitated by microgravity crystallization of TS with the Toledo Crystallization Box, we report the 2.1 Å joint X-ray/neutron (XN) structure of TS with PLP in the internal aldimine form. Positions of hydrogens were directly determined in both the α- and β-active sites, including PLP cofactor. The joint XN structure thus provides insight into the selective protonation of the internal aldimine and the electrostatic environment of TS necessary to understand the overall catalytic mechanism.
Conflict of interest statement
DECLARATION OF INTERESTS The authors declare no competing interests.
Figures
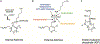
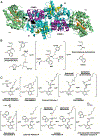
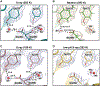
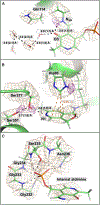
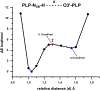
Similar articles
-
Microgravity crystallization of perdeuterated tryptophan synthase for neutron diffraction.NPJ Microgravity. 2022 May 4;8(1):13. doi: 10.1038/s41526-022-00199-3. NPJ Microgravity. 2022. PMID: 35508463 Free PMC article.
-
Pyridoxal 5'-phosphate dependent reactions: Analyzing the mechanism of aspartate aminotransferase.Methods Enzymol. 2020;634:333-359. doi: 10.1016/bs.mie.2020.01.009. Epub 2020 Feb 13. Methods Enzymol. 2020. PMID: 32093839 Review.
-
Molecular evolution of B6 enzymes: binding of pyridoxal-5'-phosphate and Lys41Arg substitution turn ribonuclease A into a model B6 protoenzyme.BMC Biochem. 2008 Jun 19;9:17. doi: 10.1186/1471-2091-9-17. BMC Biochem. 2008. PMID: 18565210 Free PMC article.
-
Direct visualization of critical hydrogen atoms in a pyridoxal 5'-phosphate enzyme.Nat Commun. 2017 Oct 16;8(1):955. doi: 10.1038/s41467-017-01060-y. Nat Commun. 2017. PMID: 29038582 Free PMC article.
-
Biomedical aspects of pyridoxal 5'-phosphate availability.Front Biosci (Elite Ed). 2012 Jan 1;4(3):897-913. doi: 10.2741/E428. Front Biosci (Elite Ed). 2012. PMID: 22201923 Review.
Cited by
-
Universality of critical active site glutamate as an acid-base catalyst in serine hydroxymethyltransferase function.Chem Sci. 2024 Jul 3;15(32):12827-12844. doi: 10.1039/d4sc03187c. eCollection 2024 Aug 14. Chem Sci. 2024. PMID: 39148791 Free PMC article.
References
Grants and funding
LinkOut - more resources
Full Text Sources
Research Materials