This is a preprint.
A Human Brain Map of Mitochondrial Respiratory Capacity and Diversity
- PMID: 38562777
- PMCID: PMC10984021
- DOI: 10.21203/rs.3.rs-4047706/v1
A Human Brain Map of Mitochondrial Respiratory Capacity and Diversity
Abstract
Mitochondrial oxidative phosphorylation (OxPhos) powers brain activity1,2, and mitochondrial defects are linked to neurodegenerative and neuropsychiatric disorders3,4, underscoring the need to define the brain's molecular energetic landscape5-10. To bridge the cognitive neuroscience and cell biology scale gap, we developed a physical voxelization approach to partition a frozen human coronal hemisphere section into 703 voxels comparable to neuroimaging resolution (3×3×3 mm). In each cortical and subcortical brain voxel, we profiled mitochondrial phenotypes including OxPhos enzyme activities, mitochondrial DNA and volume density, and mitochondria-specific respiratory capacity. We show that the human brain contains a diversity of mitochondrial phenotypes driven by both topology and cell types. Compared to white matter, grey matter contains >50% more mitochondria. We show that the more abundant grey matter mitochondria also are biochemically optimized for energy transformation, particularly among recently evolved cortical brain regions. Scaling these data to the whole brain, we created a backward linear regression model integrating several neuroimaging modalities11, thereby generating a brain-wide map of mitochondrial distribution and specialization that predicts mitochondrial characteristics in an independent brain region of the same donor brain. This new approach and the resulting MitoBrainMap of mitochondrial phenotypes provide a foundation for exploring the molecular energetic landscape that enables normal brain functions, relating it to neuroimaging data, and defining the subcellular basis for regionalized brain processes relevant to neuropsychiatric and neurodegenerative disorders.
Keywords: MRI; OxPhos; anatomical mapping; brain voxelization; mitochondria; neuroimaging; phenotyping; single-cell RNA sequencing.
Conflict of interest statement
Financial competing interests The authors have no competing interests to declare.
Figures
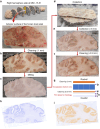
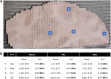
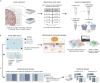
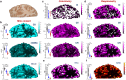
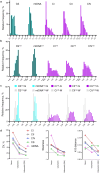
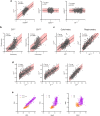
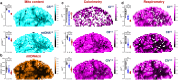
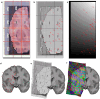
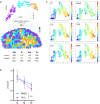
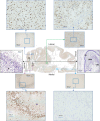
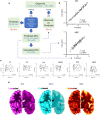
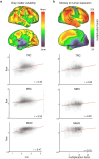
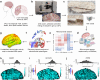
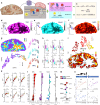
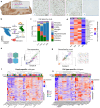
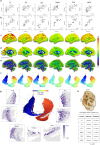
Similar articles
-
A Human Brain Map of Mitochondrial Respiratory Capacity and Diversity.bioRxiv [Preprint]. 2024 Mar 7:2024.03.05.583623. doi: 10.1101/2024.03.05.583623. bioRxiv. 2024. PMID: 38496679 Free PMC article. Preprint.
-
Anatomic & metabolic brain markers of the m.3243A>G mutation: A multi-parametric 7T MRI study.Neuroimage Clin. 2018 Jan 31;18:231-244. doi: 10.1016/j.nicl.2018.01.017. eCollection 2018. Neuroimage Clin. 2018. PMID: 29868447 Free PMC article.
-
High-Dimensional Mapping of Cognition to the Brain Using Voxel-Based Morphometry and Subcortical Shape Analysis.J Alzheimers Dis. 2019;71(1):141-152. doi: 10.3233/JAD-181297. J Alzheimers Dis. 2019. PMID: 31356202
-
Emerging roles of brain metabolism in cognitive impairment and neuropsychiatric disorders.Neurosci Biobehav Rev. 2022 Nov;142:104892. doi: 10.1016/j.neubiorev.2022.104892. Epub 2022 Sep 28. Neurosci Biobehav Rev. 2022. PMID: 36181925 Review.
-
Exploring mitochondrial system properties of neurodegenerative diseases through interactome mapping.J Proteomics. 2014 Apr 4;100:8-24. doi: 10.1016/j.jprot.2013.11.008. Epub 2013 Nov 18. J Proteomics. 2014. PMID: 24262152 Review.
References
-
- Zhang D. & Raichle M. E. Disease and the brain’s dark energy. Nat. Rev. Neurol. 6, 15–28 (2010). - PubMed
Publication types
Grants and funding
LinkOut - more resources
Full Text Sources
Miscellaneous