Microfluidic approaches for producing lipid-based nanoparticles for drug delivery applications
- PMID: 38505779
- PMCID: PMC10903496
- DOI: 10.1063/5.0150345
Microfluidic approaches for producing lipid-based nanoparticles for drug delivery applications
Abstract
The importance of drug delivery for disease treatment is supported by a vast literature and increasing ongoing clinical studies. Several categories of nano-based drug delivery systems have been considered in recent years, among which lipid-based nanomedicines, both artificial and cell-derived, remain the most approved. The best artificial systems in terms of biocompatibility and low toxicity are liposomes, as they are composed of phospholipids and cholesterol, the main components of cell membranes. Extracellular vesicles-biological nanoparticles released from cells-while resembling liposomes in size, shape, and structure, have a more complex composition with up to hundreds of different types of lipids, proteins, and carbohydrates in their membranes, as well as an internal cargo. Although nanoparticle technologies have revolutionized drug delivery by enabling passive and active targeting, increased stability, improved solubilization capacity, and reduced dose and adverse effects, the clinical translation remains challenging due to manufacturing limitations such as laborious and time-consuming procedures and high batch-to-batch variability. A sea change occurred when microfluidic strategies were employed, offering advantages in terms of precise particle handling, simplified workflows, higher sensitivity and specificity, and good reproducibility and stability over bulk methods. This review examines scientific advances in the microfluidics-mediated production of lipid-based nanoparticles for therapeutic applications. We will discuss the preparation of liposomes using both hydrodynamic focusing of microfluidic flow and mixing by herringbone and staggered baffle micromixers. Then, an overview on microfluidic approaches for producing extracellular vesicles and extracellular vesicles-mimetics for therapeutic applications will describe microfluidic extrusion, surface engineering, sonication, electroporation, nanoporation, and mixing. Finally, we will outline the challenges, opportunities, and future directions of microfluidic investigation of lipid-based nanoparticles in the clinic.
© 2023 Author(s).
Conflict of interest statement
The authors have no conflicts to disclose.
Figures
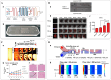
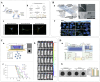
Similar articles
-
Microfluidic Manufacture of Lipid-Based Nanomedicines.Pharmaceutics. 2022 Sep 14;14(9):1940. doi: 10.3390/pharmaceutics14091940. Pharmaceutics. 2022. PMID: 36145688 Free PMC article. Review.
-
Microfluidic synthesis of lipid-based nanoparticles for drug delivery: recent advances and opportunities.Lab Chip. 2024 Feb 27;24(5):1154-1174. doi: 10.1039/d3lc00821e. Lab Chip. 2024. PMID: 38165786 Review.
-
Advances in microfluidics for lipid nanoparticles and extracellular vesicles and applications in drug delivery systems.Adv Drug Deliv Rev. 2018 Mar 15;128:84-100. doi: 10.1016/j.addr.2018.03.008. Epub 2018 Mar 19. Adv Drug Deliv Rev. 2018. PMID: 29567396 Review.
-
Development of a Microfluidic-Based Post-Treatment Process for Size-Controlled Lipid Nanoparticles and Application to siRNA Delivery.ACS Appl Mater Interfaces. 2020 Jul 29;12(30):34011-34020. doi: 10.1021/acsami.0c05489. Epub 2020 Jul 15. ACS Appl Mater Interfaces. 2020. PMID: 32667806
-
Microfluidic methods for production of liposomes.Methods Enzymol. 2009;465:129-41. doi: 10.1016/S0076-6879(09)65007-2. Methods Enzymol. 2009. PMID: 19913165 Free PMC article.
Cited by
-
Archaeosomes for Oral Drug Delivery: From Continuous Microfluidics Production to Powdered Formulations.Pharmaceutics. 2024 May 23;16(6):694. doi: 10.3390/pharmaceutics16060694. Pharmaceutics. 2024. PMID: 38931818 Free PMC article.
-
Extracellular vesicles as nanotheranostic platforms for targeted neurological disorder interventions.Nano Converg. 2024 May 13;11(1):19. doi: 10.1186/s40580-024-00426-5. Nano Converg. 2024. PMID: 38739358 Free PMC article. Review.
References
-
- Belliveau, N. M. , Huft, J. , Lin, P. J. , Chen, S. , Leung, A. K. , Leaver, T. J. , Wild, A. W. , Lee, J. B. , Taylor, R. J. , Tam, Y. K. , Hansen, C. L. , and Cullis, P. R. , “ Microfluidic synthesis of highly potent limit-size lipid nanoparticles for in vivo delivery of siRNA,” Mol. Ther. Nucl. Acids 1(8), e37 (2012).10.1038/mtna.2012.28 - DOI - PMC - PubMed
Publication types
LinkOut - more resources
Full Text Sources