Highly oriented hydrogels for tissue regeneration: design strategies, cellular mechanisms, and biomedical applications
- PMID: 38505623
- PMCID: PMC10945336
- DOI: 10.7150/thno.89493
Highly oriented hydrogels for tissue regeneration: design strategies, cellular mechanisms, and biomedical applications
Abstract
Many human tissues exhibit a highly oriented architecture that confers them with distinct mechanical properties, enabling adaptation to diverse and challenging environments. Hydrogels, with their water-rich "soft and wet" structure, have emerged as promising biomimetic materials in tissue engineering for repairing and replacing damaged tissues and organs. Highly oriented hydrogels can especially emulate the structural orientation found in human tissue, exhibiting unique physiological functions and properties absent in traditional homogeneous isotropic hydrogels. The design and preparation of highly oriented hydrogels involve strategies like including hydrogels with highly oriented nanofillers, polymer-chain networks, void channels, and microfabricated structures. Understanding the specific mechanism of action of how these highly oriented hydrogels affect cell behavior and their biological applications for repairing highly oriented tissues such as the cornea, skin, skeletal muscle, tendon, ligament, cartilage, bone, blood vessels, heart, etc., requires further exploration and generalization. Therefore, this review aims to fill that gap by focusing on the design strategy of highly oriented hydrogels and their application in the field of tissue engineering. Furthermore, we provide a detailed discussion on the application of highly oriented hydrogels in various tissues and organs and the mechanisms through which highly oriented structures influence cell behavior.
© The author(s).
Conflict of interest statement
Competing Interests: The authors have declared that no competing interest exists.
Figures
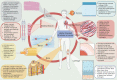

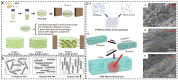
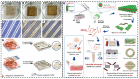
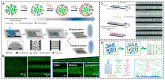
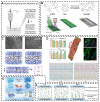
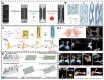
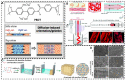
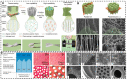
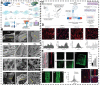
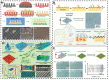
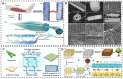
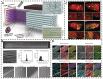
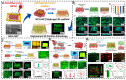
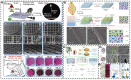
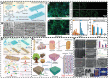
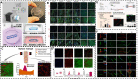
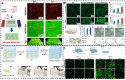
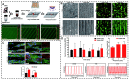
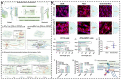
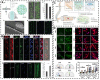
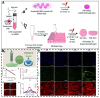
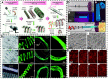
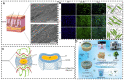
Similar articles
-
Toward the development of biomimetic injectable and macroporous biohydrogels for regenerative medicine.Adv Colloid Interface Sci. 2017 Sep;247:589-609. doi: 10.1016/j.cis.2017.07.012. Epub 2017 Jul 16. Adv Colloid Interface Sci. 2017. PMID: 28754381 Review.
-
Magnetically anisotropic hydrogels for tissue engineering.Biomater Sci. 2023 Sep 26;11(19):6384-6402. doi: 10.1039/d3bm00744h. Biomater Sci. 2023. PMID: 37552036 Review.
-
Biomimetic Hydrogels as the Inductive Endochondral Ossification Template for Promoting Bone Regeneration.Adv Healthc Mater. 2024 Jul;13(18):e2303532. doi: 10.1002/adhm.202303532. Epub 2024 May 25. Adv Healthc Mater. 2024. PMID: 38108565 Review.
-
Nanocellulose-based hydrogels as versatile materials with interesting functional properties for tissue engineering applications.J Mater Chem B. 2024 Aug 14;12(32):7692-7759. doi: 10.1039/d4tb00397g. J Mater Chem B. 2024. PMID: 38805188 Review.
-
Leveling Up Hydrogels: Hybrid Systems in Tissue Engineering.Trends Biotechnol. 2020 Mar;38(3):292-315. doi: 10.1016/j.tibtech.2019.09.004. Epub 2019 Nov 29. Trends Biotechnol. 2020. PMID: 31787346 Review.
Cited by
-
Correction to "Nanofibrous Microspheres: A Biomimetic Platform for Bone Tissue Regeneration".ACS Appl Bio Mater. 2024 Sep 16;7(9):6325-6331. doi: 10.1021/acsabm.4c01057. Epub 2024 Aug 20. ACS Appl Bio Mater. 2024. PMID: 39162584 Free PMC article. No abstract available.
-
Nanofibrous Microspheres: A Biomimetic Platform for Bone Tissue Regeneration.ACS Appl Bio Mater. 2024 Jul 15;7(7):4270-4292. doi: 10.1021/acsabm.4c00613. Epub 2024 Jul 1. ACS Appl Bio Mater. 2024. PMID: 38950103 Free PMC article. Review.
-
Fabrication of 3D Biomimetic Smooth Muscle Using Magnetic Induction and Bioprinting for Tissue Regeneration.Biomater Res. 2024 Sep 9;28:0076. doi: 10.34133/bmr.0076. eCollection 2024. Biomater Res. 2024. PMID: 39253032 Free PMC article.
References
-
- Langer R, Vacanti JP. Tissue engineering. Science. 1993;260:920–6. - PubMed
-
- Gong JP, Katsuyama Y, Kurokawa T, Osada Y. Double-network hydrogels with extremely high mechanical strength. Adv Mater. 2003;15:1155–8.
Publication types
MeSH terms
Substances
LinkOut - more resources
Full Text Sources
Miscellaneous