This is a preprint.
Lipid osmosis, membrane tension, and other mechanochemical driving forces of lipid flow
- PMID: 38260424
- PMCID: PMC10802412
- DOI: 10.1101/2024.01.08.574656
Lipid osmosis, membrane tension, and other mechanochemical driving forces of lipid flow
Update in
-
Lipid osmosis, membrane tension, and other mechanochemical driving forces of lipid flow.Curr Opin Cell Biol. 2024 Jun;88:102377. doi: 10.1016/j.ceb.2024.102377. Epub 2024 May 31. Curr Opin Cell Biol. 2024. PMID: 38823338 Review.
Abstract
Nonvesicular lipid transport among different membranes or membrane domains plays crucial roles in lipid homeostasis and organelle biogenesis. However, the forces that drive such lipid transport are not well understood. We propose that lipids tend to flow towards the membrane area with a higher membrane protein density in a process termed lipid osmosis. This process lowers the membrane tension in the area, resulting in a membrane tension difference called osmotic membrane tension. We examine the thermodynamic basis and experimental evidence of lipid osmosis and osmotic membrane tension. We predict that lipid osmosis can drive bulk lipid flows between different membrane regions through lipid transfer proteins, scramblases, or other similar barriers that selectively pass lipids but not membrane proteins. We also speculate on the biological functions of lipid osmosis. Finally, we explore other driving forces for lipid transfer and describe potential methods and systems to further test our theory.
Conflict of interest statement
Conflict of interest The authors declare no conflict of interest.
Figures
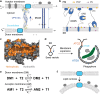
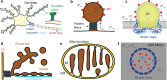
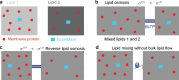
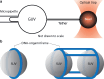
Similar articles
-
Depressing time: Waiting, melancholia, and the psychoanalytic practice of care.In: Kirtsoglou E, Simpson B, editors. The Time of Anthropology: Studies of Contemporary Chronopolitics. Abingdon: Routledge; 2020. Chapter 5. In: Kirtsoglou E, Simpson B, editors. The Time of Anthropology: Studies of Contemporary Chronopolitics. Abingdon: Routledge; 2020. Chapter 5. PMID: 36137063 Free Books & Documents. Review.
-
Dynamic Field Theory of Executive Function: Identifying Early Neurocognitive Markers.Monogr Soc Res Child Dev. 2024 Dec;89(3):7-109. doi: 10.1111/mono.12478. Monogr Soc Res Child Dev. 2024. PMID: 39628288 Free PMC article.
-
Enabling Systemic Identification and Functionality Profiling for Cdc42 Homeostatic Modulators.bioRxiv [Preprint]. 2024 Jan 8:2024.01.05.574351. doi: 10.1101/2024.01.05.574351. bioRxiv. 2024. Update in: Commun Chem. 2024 Nov 19;7(1):271. doi: 10.1038/s42004-024-01352-7. PMID: 38260445 Free PMC article. Updated. Preprint.
-
Australia in 2030: what is our path to health for all?Med J Aust. 2021 May;214 Suppl 8:S5-S40. doi: 10.5694/mja2.51020. Med J Aust. 2021. PMID: 33934362
-
Strategies to improve smoking cessation rates in primary care.Cochrane Database Syst Rev. 2021 Sep 6;9(9):CD011556. doi: 10.1002/14651858.CD011556.pub2. Cochrane Database Syst Rev. 2021. PMID: 34693994 Free PMC article. Review.
References
-
- Hanna M, Guillen-Samander A, De Camilli P: RBG motif bridge-like lipid transport proteins: structure, functions, and open questions. Annu. Rev. Cell. Dev. Biol. 2023, 39:409–434 - PubMed
-
- Wong LH, Gatta AT, Levine TP: Lipid transfer proteins: the lipid commute via shuttles, bridges and tubes. Nat. Rev. Mol. Cell Bio. 2019, 20:85–101 - PubMed
Publication types
Grants and funding
LinkOut - more resources
Full Text Sources