Structure of saguaro cactus virus 3' translational enhancer mimics 5' cap for eIF4E binding
- PMID: 38241435
- PMCID: PMC10823258
- DOI: 10.1073/pnas.2313677121
Structure of saguaro cactus virus 3' translational enhancer mimics 5' cap for eIF4E binding
Abstract
The genomes of several plant viruses contain RNA structures at their 3' ends called cap-independent translation enhancers (CITEs) that bind the host protein factors such as mRNA 5' cap-binding protein eIF4E for promoting cap-independent genome translation. However, the structural basis of such 5' cap-binding protein recognition by the uncapped RNA remains largely unknown. Here, we have determined the crystal structure of a 3' CITE, panicum mosaic virus-like translation enhancer (PTE) from the saguaro cactus virus (SCV), using a Fab crystallization chaperone. The PTE RNA folds into a three-way junction architecture with a pseudoknot between the purine-rich R domain and pyrimidine-rich Y domain, which organizes the overall structure to protrude out a specific guanine nucleotide, G18, from the R domain that comprises a major interaction site for the eIF4E binding. The superimposable crystal structures of the wild-type, G18A, G18C, and G18U mutants suggest that the PTE scaffold is preorganized with the flipped-out G18 ready to dock into the eIF4E 5' cap-binding pocket. The binding studies with wheat and human eIF4Es using gel electrophoresis and isothermal titration calorimetry, and molecular docking computation for the PTE-eIF4E complex demonstrated that the PTE structure essentially mimics the mRNA 5' cap for eIF4E binding. Such 5' cap mimicry by the uncapped and structured viral RNA highlights how viruses can exploit RNA structures to mimic the host protein-binding partners and bypass the canonical mechanisms for their genome translation, providing opportunities for a better understanding of virus-host interactions and non-canonical translation mechanisms found in many pathogenic RNA viruses.
Keywords: 3′ cap-independent translation enhancers; RNA crystallography; RNA–eIF4E interactions; crystallization chaperones; viral RNAs.
Conflict of interest statement
Competing interests statement:The authors declare no competing interest.
Figures
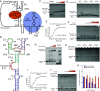
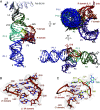
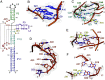
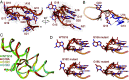
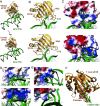
Similar articles
-
Structure of a viral cap-independent translation element that functions via high affinity binding to the eIF4E subunit of eIF4F.J Biol Chem. 2009 May 22;284(21):14189-202. doi: 10.1074/jbc.M808841200. Epub 2009 Mar 10. J Biol Chem. 2009. PMID: 19276085 Free PMC article.
-
Crystal structure of a cap-independent translation enhancer RNA.Nucleic Acids Res. 2023 Sep 8;51(16):8891-8907. doi: 10.1093/nar/gkad649. Nucleic Acids Res. 2023. PMID: 37548413 Free PMC article.
-
The cap-binding translation initiation factor, eIF4E, binds a pseudoknot in a viral cap-independent translation element.Structure. 2011 Jun 8;19(6):868-80. doi: 10.1016/j.str.2011.03.013. Structure. 2011. PMID: 21645857 Free PMC article.
-
Contrasting mechanisms of regulating translation of specific Drosophila germline mRNAs at the level of 5'-cap structure binding.Biochem Soc Trans. 2005 Dec;33(Pt 6):1544-6. doi: 10.1042/BST0331544. Biochem Soc Trans. 2005. PMID: 16246166 Review.
-
Cap-dependent translation initiation factor eIF4E: an emerging anticancer drug target.Med Res Rev. 2012 Jul;32(4):786-814. doi: 10.1002/med.21260. Epub 2012 Apr 11. Med Res Rev. 2012. PMID: 22495651 Free PMC article. Review.
Cited by
-
Host-like RNA Elements Regulate Virus Translation.Viruses. 2024 Mar 20;16(3):468. doi: 10.3390/v16030468. Viruses. 2024. PMID: 38543832 Free PMC article. Review.
-
mRNA vaccines in tumor targeted therapy: mechanism, clinical application, and development trends.Biomark Res. 2024 Aug 31;12(1):93. doi: 10.1186/s40364-024-00644-3. Biomark Res. 2024. PMID: 39217377 Free PMC article. Review.
References
MeSH terms
Substances
Grants and funding
LinkOut - more resources
Full Text Sources
Miscellaneous