Contralateral Astrocyte Response to Acute Optic Nerve Damage Is Mitigated by PANX1 Channel Activity
- PMID: 37958624
- PMCID: PMC10647301
- DOI: 10.3390/ijms242115641
Contralateral Astrocyte Response to Acute Optic Nerve Damage Is Mitigated by PANX1 Channel Activity
Abstract
Glial reactivity is considered a hallmark of damage-induced innate immune responses in the central nervous system. In the visual system, unilateral optic nerve damage elicits dramatic glial reactivity in the retina directly affected by the lesion and a similar, albeit more modest, effect in the contralateral eye. Evaluation of astrocyte changes in a mouse model of optic nerve crush indicates that astrocyte reactivity, as a function of retinal coverage and cellular hypertrophy, occurs within both the experimental and contralateral retinas, although the hypertrophic response of the astrocytes in the contralateral eyes is delayed for at least 24 h. Evaluation of astrocytic reactivity as a function of Gfap expression indicates a similar, muted but significant, response in contralateral eyes. This constrained glial response is completely negated by conditional knock out of Panx1 in both astrocytes and Müller cells. Further studies are required to identify if this is an autocrine or a paracrine suppression of astroglial reactivity.
Keywords: Müller cell; PANX1 hemichannels; astrocyte; contralateral retina; unilateral optic nerve damage.
Conflict of interest statement
The authors declare no conflict of interest.
Figures
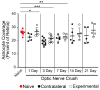
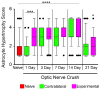
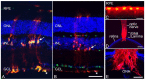
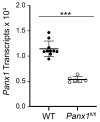
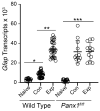
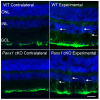
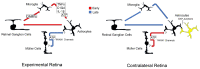
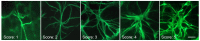
Similar articles
-
Unilateral injury to the adult rat optic nerve causes multiple cellular responses in the contralateral site.J Neurobiol. 1999 Jan;38(1):116-28. doi: 10.1002/(sici)1097-4695(199901)38:1<116::aid-neu9>3.0.co;2-f. J Neurobiol. 1999. PMID: 10027567
-
Glioprotection of Retinal Astrocytes After Intravitreal Administration of Memantine in the Mouse Optic Nerve Crush Model.Med Sci Monit. 2017 Mar 7;23:1173-1179. doi: 10.12659/msm.899699. Med Sci Monit. 2017. PMID: 28265105 Free PMC article.
-
Retinal glial responses to optic nerve crush are attenuated in Bax-deficient mice and modulated by purinergic signaling pathways.J Neuroinflammation. 2016 Apr 28;13(1):93. doi: 10.1186/s12974-016-0558-y. J Neuroinflammation. 2016. PMID: 27126275 Free PMC article.
-
Insight into astrocyte activation after optic nerve injury.J Neurosci Res. 2015 Apr;93(4):539-48. doi: 10.1002/jnr.23487. Epub 2014 Sep 26. J Neurosci Res. 2015. PMID: 25257183 Review.
-
Astrocyte Immune Functions and Glaucoma.Int J Mol Sci. 2023 Feb 1;24(3):2747. doi: 10.3390/ijms24032747. Int J Mol Sci. 2023. PMID: 36769067 Free PMC article. Review.
Cited by
-
Increased Pan-Type, A1-Type, and A2-Type Astrocyte Activation and Upstream Inflammatory Markers Are Induced by the P2X7 Receptor.Int J Mol Sci. 2024 Aug 13;25(16):8784. doi: 10.3390/ijms25168784. Int J Mol Sci. 2024. PMID: 39201471 Free PMC article.
-
DAMPs Drive Fibroinflammatory Changes in the Glaucomatous ONH.Invest Ophthalmol Vis Sci. 2024 Oct 1;65(12):13. doi: 10.1167/iovs.65.12.13. Invest Ophthalmol Vis Sci. 2024. PMID: 39382882 Free PMC article.
References
-
- Colombo G., Cubero R.J.A., Kanari L., Venturino A., Schulz R., Scolamiero M., Agerberg J., Mathys H., Tsai L.-H., Chachólski W., et al. A tool for mapping microglial morphology, morphOMICS, reveals brain-region and sex-dependent phenotypes. Nat. Neurosci. 2022;25:1379–1393. doi: 10.1038/s41593-022-01167-6. - DOI - PMC - PubMed
MeSH terms
Substances
Grants and funding
LinkOut - more resources
Full Text Sources
Medical
Molecular Biology Databases
Miscellaneous