Driving forces of the complex formation between highly charged disordered proteins
- PMID: 37796987
- PMCID: PMC10576128
- DOI: 10.1073/pnas.2304036120
Driving forces of the complex formation between highly charged disordered proteins
Abstract
Highly disordered complexes between oppositely charged intrinsically disordered proteins present a new paradigm of biomolecular interactions. Here, we investigate the driving forces of such interactions for the example of the highly positively charged linker histone H1 and its highly negatively charged chaperone, prothymosin α (ProTα). Temperature-dependent single-molecule Förster resonance energy transfer (FRET) experiments and isothermal titration calorimetry reveal ProTα-H1 binding to be enthalpically unfavorable, and salt-dependent affinity measurements suggest counterion release entropy to be an important thermodynamic driving force. Using single-molecule FRET, we also identify ternary complexes between ProTα and H1 in addition to the heterodimer at equilibrium and show how they contribute to the thermodynamics observed in ensemble experiments. Finally, we explain the observed thermodynamics quantitatively with a mean-field polyelectrolyte theory that treats counterion release explicitly. ProTα-H1 complex formation resembles the interactions between synthetic polyelectrolytes, and the underlying principles are likely to be of broad relevance for interactions between charged biomolecules in general.
Keywords: intrinsically disordered proteins; polyelectrolyte complexation; protein binding; single-molecule spectroscopy.
Conflict of interest statement
The authors declare no competing interest.
Figures
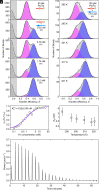
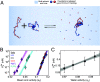
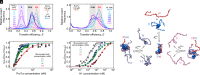
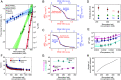
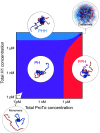
Similar articles
-
Affinity of disordered protein complexes is modulated by entropy-energy reinforcement.Proc Natl Acad Sci U S A. 2022 Jun 28;119(26):e2120456119. doi: 10.1073/pnas.2120456119. Epub 2022 Jun 21. Proc Natl Acad Sci U S A. 2022. PMID: 35727975 Free PMC article.
-
Binding Affinity and Function of the Extremely Disordered Protein Complex Containing Human Linker Histone H1.0 and Its Chaperone ProTα.Biochemistry. 2018 Dec 4;57(48):6645-6648. doi: 10.1021/acs.biochem.8b01075. Epub 2018 Nov 19. Biochemistry. 2018. PMID: 30430826 Free PMC article.
-
Polyelectrolyte interactions enable rapid association and dissociation in high-affinity disordered protein complexes.Nat Commun. 2020 Nov 12;11(1):5736. doi: 10.1038/s41467-020-18859-x. Nat Commun. 2020. PMID: 33184256 Free PMC article.
-
Interaction of Proteins with Polyelectrolytes: Comparison of Theory to Experiment.Langmuir. 2019 Apr 23;35(16):5373-5391. doi: 10.1021/acs.langmuir.8b01802. Epub 2018 Aug 24. Langmuir. 2019. PMID: 30095921 Review.
-
Binding without folding - the biomolecular function of disordered polyelectrolyte complexes.Curr Opin Struct Biol. 2020 Feb;60:66-76. doi: 10.1016/j.sbi.2019.12.006. Epub 2019 Dec 23. Curr Opin Struct Biol. 2020. PMID: 31874413 Review.
Cited by
-
Multifunctional biomolecular corona-inspired nanoremediation of antibiotic residues.Proc Natl Acad Sci U S A. 2024 Sep 3;121(36):e2409955121. doi: 10.1073/pnas.2409955121. Epub 2024 Aug 27. Proc Natl Acad Sci U S A. 2024. PMID: 39190351
-
Beyond monopole electrostatics in regulating conformations of intrinsically disordered proteins.PNAS Nexus. 2024 Aug 27;3(9):pgae367. doi: 10.1093/pnasnexus/pgae367. eCollection 2024 Sep. PNAS Nexus. 2024. PMID: 39253398 Free PMC article.
-
Unlocking the electrochemical functions of biomolecular condensates.Nat Chem Biol. 2024 Nov;20(11):1420-1433. doi: 10.1038/s41589-024-01717-y. Epub 2024 Sep 26. Nat Chem Biol. 2024. PMID: 39327453 Review.
-
Biomolecular condensates regulate cellular electrochemical equilibria.Cell. 2024 Oct 17;187(21):5951-5966.e18. doi: 10.1016/j.cell.2024.08.018. Epub 2024 Sep 10. Cell. 2024. PMID: 39260373
-
Biomolecular condensates form spatially inhomogeneous network fluids.Nat Commun. 2024 Apr 22;15(1):3413. doi: 10.1038/s41467-024-47602-z. Nat Commun. 2024. PMID: 38649740 Free PMC article.
References
-
- Dunker A. K., Cortese M. S., Romero P., Iakoucheva L. M., Uversky V. N., Flexible nets. The roles of intrinsic disorder in protein interaction networks. FEBS J. 272, 5129–5148 (2005). - PubMed
-
- Tompa P., Fuxreiter M., Fuzzy complexes: Polymorphism and structural disorder in protein-protein interactions. Trends Biochem. Sci. 33, 2–8 (2008). - PubMed
Publication types
MeSH terms
Substances
LinkOut - more resources
Full Text Sources