Therapeutic Oligonucleotides: An Outlook on Chemical Strategies to Improve Endosomal Trafficking
- PMID: 37759475
- PMCID: PMC10527716
- DOI: 10.3390/cells12182253
Therapeutic Oligonucleotides: An Outlook on Chemical Strategies to Improve Endosomal Trafficking
Abstract
The potential of oligonucleotide therapeutics is undeniable as more than 15 drugs have been approved to treat various diseases in the liver, central nervous system (CNS), and muscles. However, achieving effective delivery of oligonucleotide therapeutics to specific tissues still remains a major challenge, limiting their widespread use. Chemical modifications play a crucial role to overcome biological barriers to enable efficient oligonucleotide delivery to the tissues/cells of interest. They provide oligonucleotide metabolic stability and confer favourable pharmacokinetic/pharmacodynamic properties. This review focuses on the various chemical approaches implicated in mitigating the delivery problem of oligonucleotides and their limitations. It highlights the importance of linkers in designing oligonucleotide conjugates and discusses their potential role in escaping the endosomal barrier, a bottleneck in the development of oligonucleotide therapeutics.
Keywords: antisense oligonucleotides (ASOs); cleavable linkers; endosomal escape; endosomolytic agents; linker chemistry; non-cleavable linkers; oligonucleotide conjugates; oligonucleotide delivery; oligonucleotide therapeutics; pH-sensitive linkers; siRNA.
Conflict of interest statement
The authors declare no conflict of interest.
Figures
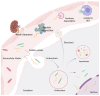
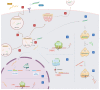
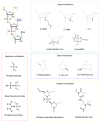
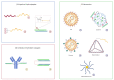
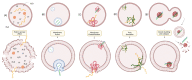
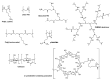
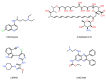
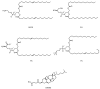
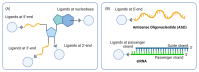
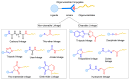
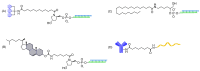
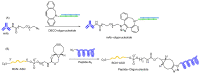
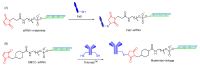
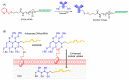
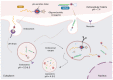
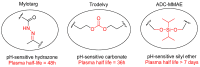
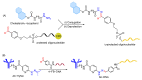
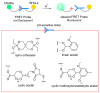
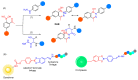
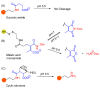
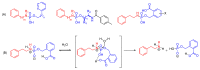
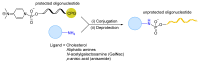
Similar articles
-
Hyaluronan-based delivery of therapeutic oligonucleotides for treatment of human diseases.Expert Opin Drug Deliv. 2019 Jun;16(6):621-637. doi: 10.1080/17425247.2019.1617693. Epub 2019 May 20. Expert Opin Drug Deliv. 2019. PMID: 31072142 Review.
-
Chemical Manipulation of the Endosome Trafficking Machinery: Implications for Oligonucleotide Delivery.Biomedicines. 2021 May 5;9(5):512. doi: 10.3390/biomedicines9050512. Biomedicines. 2021. PMID: 34063104 Free PMC article. Review.
-
Endosomal escape cell-penetrating peptides significantly enhance pharmacological effectiveness and CNS activity of systemically administered antisense oligonucleotides.Int J Pharm. 2021 Apr 15;599:120398. doi: 10.1016/j.ijpharm.2021.120398. Epub 2021 Feb 26. Int J Pharm. 2021. PMID: 33640427
-
Site Selective Antibody-Oligonucleotide Conjugation via Microbial Transglutaminase.Molecules. 2019 Sep 10;24(18):3287. doi: 10.3390/molecules24183287. Molecules. 2019. PMID: 31509944 Free PMC article.
-
Simultaneous inhibition of endocytic recycling and lysosomal fusion sensitizes cells and tissues to oligonucleotide therapeutics.Nucleic Acids Res. 2023 Feb 28;51(4):1583-1599. doi: 10.1093/nar/gkad023. Nucleic Acids Res. 2023. PMID: 36727438 Free PMC article.
Cited by
-
Thiol-Specific Linkers for the Synthesis of Oligonucleotide Conjugates via Metal-Free Thiol-Ene Click Reaction.Bioconjug Chem. 2024 Sep 12;35(10):1553-67. doi: 10.1021/acs.bioconjchem.4c00336. Online ahead of print. Bioconjug Chem. 2024. PMID: 39264307 Free PMC article.
References
Publication types
MeSH terms
Substances
Grants and funding
LinkOut - more resources
Full Text Sources