Tomosyn affects dense core vesicle composition but not exocytosis in mammalian neurons
- PMID: 37695731
- PMCID: PMC10495110
- DOI: 10.7554/eLife.85561
Tomosyn affects dense core vesicle composition but not exocytosis in mammalian neurons
Abstract
Tomosyn is a large, non-canonical SNARE protein proposed to act as an inhibitor of SNARE complex formation in the exocytosis of secretory vesicles. In the brain, tomosyn inhibits the fusion of synaptic vesicles (SVs), whereas its role in the fusion of neuropeptide-containing dense core vesicles (DCVs) is unknown. Here, we addressed this question using a new mouse model with a conditional deletion of tomosyn (Stxbp5) and its paralogue tomosyn-2 (Stxbp5l). We monitored DCV exocytosis at single vesicle resolution in tomosyn-deficient primary neurons using a validated pHluorin-based assay. Surprisingly, loss of tomosyns did not affect the number of DCV fusion events but resulted in a strong reduction of intracellular levels of DCV cargos, such as neuropeptide Y (NPY) and brain-derived neurotrophic factor (BDNF). BDNF levels were largely restored by re-expression of tomosyn but not by inhibition of lysosomal proteolysis. Tomosyn's SNARE domain was dispensable for the rescue. The size of the trans-Golgi network and DCVs was decreased, and the speed of DCV cargo flux through Golgi was increased in tomosyn-deficient neurons, suggesting a role for tomosyns in DCV biogenesis. Additionally, tomosyn-deficient neurons showed impaired mRNA expression of some DCV cargos, which was not restored by re-expression of tomosyn and was also observed in Cre-expressing wild-type neurons not carrying loxP sites, suggesting a direct effect of Cre recombinase on neuronal transcription. Taken together, our findings argue against an inhibitory role of tomosyns in neuronal DCV exocytosis and suggests an evolutionary conserved function of tomosyns in the packaging of secretory cargo at the Golgi.
Keywords: SNARE; cell biology; dense core vesicle; mouse; neuropeptides; neuroscience; secretion; tomosyn.
© 2023, Subkhangulova et al.
Conflict of interest statement
AS, MG, AG, Jv, AS, RT, MV No competing interests declared
Figures
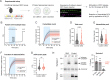
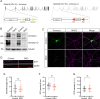
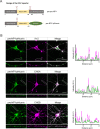
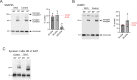
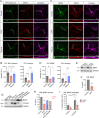
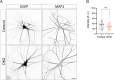
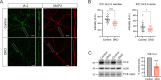
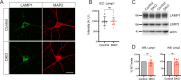
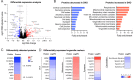
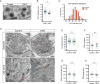
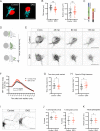
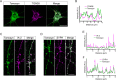
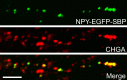
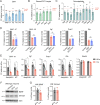
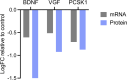
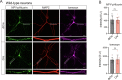
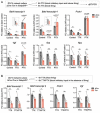
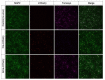
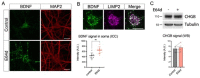
Update of
- doi: 10.1101/2022.12.18.520925
Similar articles
-
Tomosyn associates with secretory vesicles in neurons through its N- and C-terminal domains.PLoS One. 2017 Jul 26;12(7):e0180912. doi: 10.1371/journal.pone.0180912. eCollection 2017. PLoS One. 2017. PMID: 28746398 Free PMC article.
-
Rabphilin-3A negatively regulates neuropeptide release, through its SNAP25 interaction.Elife. 2024 Oct 16;13:RP95371. doi: 10.7554/eLife.95371. Elife. 2024. PMID: 39412498 Free PMC article.
-
The Phosphoprotein Synapsin Ia Regulates the Kinetics of Dense-Core Vesicle Release.J Neurosci. 2021 Mar 31;41(13):2828-2841. doi: 10.1523/JNEUROSCI.2593-19.2021. Epub 2021 Feb 25. J Neurosci. 2021. PMID: 33632727 Free PMC article.
-
TRP Channel Trafficking.In: Liedtke WB, Heller S, editors. TRP Ion Channel Function in Sensory Transduction and Cellular Signaling Cascades. Boca Raton (FL): CRC Press/Taylor & Francis; 2007. Chapter 23. In: Liedtke WB, Heller S, editors. TRP Ion Channel Function in Sensory Transduction and Cellular Signaling Cascades. Boca Raton (FL): CRC Press/Taylor & Francis; 2007. Chapter 23. PMID: 21204515 Free Books & Documents. Review.
-
Vti Proteins: Beyond Endolysosomal Trafficking.Neuroscience. 2019 Nov 10;420:32-40. doi: 10.1016/j.neuroscience.2018.11.014. Epub 2018 Nov 22. Neuroscience. 2019. PMID: 30471354 Review.
Cited by
-
Tomosyns attenuate SNARE assembly and synaptic depression by binding to VAMP2-containing template complexes.Nat Commun. 2024 Mar 26;15(1):2652. doi: 10.1038/s41467-024-46828-1. Nat Commun. 2024. PMID: 38531902 Free PMC article.
References
-
- Barak B, Williams A, Bielopolski N, Gottfried I, Okun E, Brown MA, Matti U, Rettig J, Stuenkel EL, Ashery U. Tomosyn expression pattern in the mouse hippocampus suggests both presynaptic and postsynaptic functions. Frontiers in Neuroanatomy. 2010;4:149. doi: 10.3389/fnana.2010.00149. - DOI - PMC - PubMed
-
- Bielopolski N, Lam AD, Bar-On D, Sauer M, Stuenkel EL, Ashery U. Differential interaction of tomosyn with syntaxin and SNAP25 depends on domains in the WD40 β-propeller core and determines its inhibitory activity. The Journal of Biological Chemistry. 2014;289:17087–17099. doi: 10.1074/jbc.M113.515296. - DOI - PMC - PubMed
Publication types
MeSH terms
Substances
Grants and funding
LinkOut - more resources
Full Text Sources
Miscellaneous