Rapid RGR-dependent visual pigment recycling is mediated by the RPE and specialized Müller glia
- PMID: 37585292
- PMCID: PMC10530494
- DOI: 10.1016/j.celrep.2023.112982
Rapid RGR-dependent visual pigment recycling is mediated by the RPE and specialized Müller glia
Abstract
In daylight, demand for visual chromophore (11-cis-retinal) exceeds supply by the classical visual cycle. This shortfall is compensated, in part, by the retinal G-protein-coupled receptor (RGR) photoisomerase, which is expressed in both the retinal pigment epithelium (RPE) and in Müller cells. The relative contributions of these two cellular pools of RGR to the maintenance of photoreceptor light responses are not known. Here, we use a cell-specific gene reactivation approach to elucidate the kinetics of RGR-mediated recovery of photoreceptor responses following light exposure. Electroretinographic measurements in mice with RGR expression limited to either cell type reveal that the RPE and a specialized subset of Müller glia contribute both to scotopic and photopic function. We demonstrate that 11-cis-retinal formed through photoisomerization is rapidly hydrolyzed, consistent with its role in a rapid visual pigment regeneration process. Our study shows that RGR provides a pan-retinal sink for all-trans-retinal released under sustained light conditions and supports rapid chromophore regeneration through the photic visual cycle.
Keywords: 11-cis-retinal; CP: Cell biology; CP: Neuroscience; Müller cells; chromophore; cone opsin; photic visual cycle; photoisomerization; retina; retinal pigmented epithelium; vision; visual cycle.
Copyright © 2023 The Author(s). Published by Elsevier Inc. All rights reserved.
Conflict of interest statement
Declaration of interests K.P. is a consultant to Polgenix, Inc. His relationship with Polgenix, Inc., has been reviewed and approved by the University of California, Irvine, in accordance with its conflict-of-interest policies.
Figures
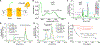
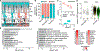
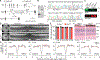
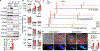
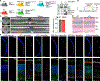
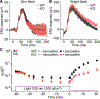
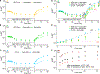
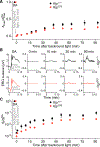
Similar articles
-
Photic generation of 11-cis-retinal in bovine retinal pigment epithelium.J Biol Chem. 2019 Dec 13;294(50):19137-19154. doi: 10.1074/jbc.RA119.011169. Epub 2019 Nov 6. J Biol Chem. 2019. PMID: 31694912 Free PMC article.
-
cis Retinol oxidation regulates photoreceptor access to the retina visual cycle and cone pigment regeneration.J Physiol. 2016 Nov 15;594(22):6753-6765. doi: 10.1113/JP272831. Epub 2016 Aug 2. J Physiol. 2016. PMID: 27385534 Free PMC article.
-
The Visual Cycle in the Inner Retina of Chicken and the Involvement of Retinal G-Protein-Coupled Receptor (RGR).Mol Neurobiol. 2017 May;54(4):2507-2517. doi: 10.1007/s12035-016-9830-5. Epub 2016 Mar 17. Mol Neurobiol. 2017. PMID: 26984602
-
Retinoids in the visual cycle: role of the retinal G protein-coupled receptor.J Lipid Res. 2021;62:100040. doi: 10.1194/jlr.TR120000850. Epub 2021 Feb 6. J Lipid Res. 2021. PMID: 32493732 Free PMC article. Review.
-
Vitamin A metabolism in rod and cone visual cycles.Annu Rev Nutr. 2012 Aug 21;32:125-45. doi: 10.1146/annurev-nutr-071811-150748. Annu Rev Nutr. 2012. PMID: 22809103 Review.
Cited by
-
Expression of proteins supporting visual function in heterobranch gastropods.J Comp Physiol A Neuroethol Sens Neural Behav Physiol. 2024 Aug 9. doi: 10.1007/s00359-024-01712-7. Online ahead of print. J Comp Physiol A Neuroethol Sens Neural Behav Physiol. 2024. PMID: 39120725
-
Cell-cell interaction in the pathogenesis of inherited retinal diseases.Front Cell Dev Biol. 2024 Mar 4;12:1332944. doi: 10.3389/fcell.2024.1332944. eCollection 2024. Front Cell Dev Biol. 2024. PMID: 38500685 Free PMC article. Review.
-
Proteopathy Linked to Exon-Skipping Isoform of RGR-Opsin Contributes to the Pathogenesis of Age-Related Macular Degeneration.Invest Ophthalmol Vis Sci. 2023 Oct 3;64(13):41. doi: 10.1167/iovs.64.13.41. Invest Ophthalmol Vis Sci. 2023. PMID: 37883094 Free PMC article.
-
Scavenging of Cation Radicals of the Visual Cycle Retinoids by Lutein, Zeaxanthin, Taurine, and Melanin.Int J Mol Sci. 2023 Dec 29;25(1):506. doi: 10.3390/ijms25010506. Int J Mol Sci. 2023. PMID: 38203675 Free PMC article.
-
Retinoid Synthesis Regulation by Retinal Cells in Health and Disease.Cells. 2024 May 18;13(10):871. doi: 10.3390/cells13100871. Cells. 2024. PMID: 38786093 Free PMC article.
References
Publication types
MeSH terms
Substances
Grants and funding
LinkOut - more resources
Full Text Sources
Molecular Biology Databases
Miscellaneous