Understanding the Rhodopsin Worldview Through Atomic Force Microscopy (AFM): Structure, Stability, and Activity Studies
- PMID: 37265335
- PMCID: PMC10908267
- DOI: 10.1002/tcr.202300113
Understanding the Rhodopsin Worldview Through Atomic Force Microscopy (AFM): Structure, Stability, and Activity Studies
Abstract
Rhodopsin is a G protein-coupled receptor (GPCR) present in the rod outer segment (ROS) of photoreceptor cells that initiates the phototransduction cascade required for scotopic vision. Due to the remarkable advancements in technological tools, the chemistry of rhodopsin has begun to unravel especially over the past few decades, but mostly at the ensemble scale. Atomic force microscopy (AFM) is a tool capable of providing critical information from a single-molecule point of view. In this regard, to bolster our understanding of rhodopsin at the nanoscale level, AFM-based imaging, force spectroscopy, and nano-indentation techniques were employed on ROS disc membranes containing rhodopsin, isolated from vertebrate species both in normal and diseased states. These AFM studies on samples from native retinal tissue have provided fundamental insights into the structure and function of rhodopsin under normal and dysfunctional states. We review here the findings from these AFM studies that provide important insights on the supramolecular organization of rhodopsin within the membrane and factors that contribute to this organization, the molecular interactions stabilizing the structure of the receptor and factors that can modify those interactions, and the mechanism underlying constitutive activity in the receptor that can cause disease.
Keywords: Atomic force microscopy (AFM); Constitutive activity; G protein-coupled receptor (GPCR); Phototransduction; Rhodopsin.
© 2023 The Authors. The Chemical Record published by The Chemical Society of Japan and Wiley-VCH GmbH.
Figures
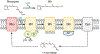
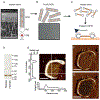
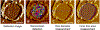
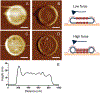
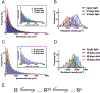
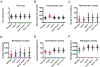
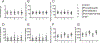
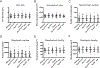
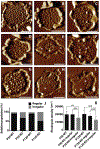
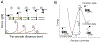
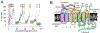
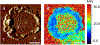
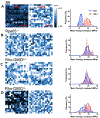
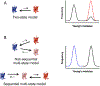
Similar articles
-
Investigating the Nanodomain Organization of Rhodopsin in Native Membranes by Atomic Force Microscopy.Methods Mol Biol. 2019;1886:61-74. doi: 10.1007/978-1-4939-8894-5_4. Methods Mol Biol. 2019. PMID: 30374862 Free PMC article.
-
Differentiating between Inactive and Active States of Rhodopsin by Atomic Force Microscopy in Native Membranes.Anal Chem. 2019 Jun 4;91(11):7226-7235. doi: 10.1021/acs.analchem.9b00546. Epub 2019 May 16. Anal Chem. 2019. PMID: 31074606 Free PMC article.
-
Nanodomain organization of rhodopsin in native human and murine rod outer segment disc membranes.Biochim Biophys Acta. 2015 Jan;1848(1 Pt A):26-34. doi: 10.1016/j.bbamem.2014.10.007. Epub 2014 Oct 12. Biochim Biophys Acta. 2015. PMID: 25305340 Free PMC article.
-
Supramolecular organization of rhodopsin in rod photoreceptor cell membranes.Pflugers Arch. 2021 Sep;473(9):1361-1376. doi: 10.1007/s00424-021-02522-5. Epub 2021 Feb 16. Pflugers Arch. 2021. PMID: 33591421 Free PMC article. Review.
-
Structure of the rhodopsin dimer: a working model for G-protein-coupled receptors.Curr Opin Struct Biol. 2006 Apr;16(2):252-9. doi: 10.1016/j.sbi.2006.03.013. Epub 2006 Mar 29. Curr Opin Struct Biol. 2006. PMID: 16567090 Review.
Cited by
-
Aggregation of rhodopsin mutants in mouse models of autosomal dominant retinitis pigmentosa.Nat Commun. 2024 Feb 16;15(1):1451. doi: 10.1038/s41467-024-45748-4. Nat Commun. 2024. PMID: 38365903 Free PMC article.
References
Publication types
MeSH terms
Substances
Grants and funding
LinkOut - more resources
Full Text Sources
Miscellaneous