Hydrogel-in-hydrogel live bioprinting for guidance and control of organoids and organotypic cultures
- PMID: 37253730
- PMCID: PMC10229611
- DOI: 10.1038/s41467-023-37953-4
Hydrogel-in-hydrogel live bioprinting for guidance and control of organoids and organotypic cultures
Abstract
Three-dimensional hydrogel-based organ-like cultures can be applied to study development, regeneration, and disease in vitro. However, the control of engineered hydrogel composition, mechanical properties and geometrical constraints tends to be restricted to the initial time of fabrication. Modulation of hydrogel characteristics over time and according to culture evolution is often not possible. Here, we overcome these limitations by developing a hydrogel-in-hydrogel live bioprinting approach that enables the dynamic fabrication of instructive hydrogel elements within pre-existing hydrogel-based organ-like cultures. This can be achieved by crosslinking photosensitive hydrogels via two-photon absorption at any time during culture. We show that instructive hydrogels guide neural axon directionality in growing organotypic spinal cords, and that hydrogel geometry and mechanical properties control differential cell migration in developing cancer organoids. Finally, we show that hydrogel constraints promote cell polarity in liver organoids, guide small intestinal organoid morphogenesis and control lung tip bifurcation according to the hydrogel composition and shape.
© 2023. The Author(s).
Conflict of interest statement
N.E. and O.G. have an equity stake in ONYEL Biotech s.r.l. A.U. and N.E. are inventors of a patent for the use of HCC- and CMM-hydrogels (patent applicant: ONYEL Biotech s.r.l.; patent number EP4138941). All other authors have no competing interests.
Figures
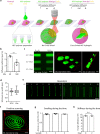
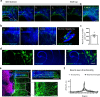
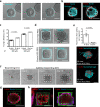
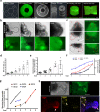
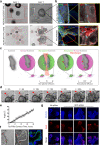
Similar articles
-
Bioprinting of Cells, Organoids and Organs-on-a-Chip Together with Hydrogels Improves Structural and Mechanical Cues.Cells. 2024 Oct 1;13(19):1638. doi: 10.3390/cells13191638. Cells. 2024. PMID: 39404401 Free PMC article. Review.
-
Bioprinting of Cell-Laden Microfiber: Can It Become a Standard Product?Adv Healthc Mater. 2019 May;8(9):e1900014. doi: 10.1002/adhm.201900014. Epub 2019 Mar 13. Adv Healthc Mater. 2019. PMID: 30866173
-
3D bioprinting of complex channels within cell-laden hydrogels.Acta Biomater. 2019 Sep 1;95:214-224. doi: 10.1016/j.actbio.2019.02.038. Epub 2019 Mar 1. Acta Biomater. 2019. PMID: 30831327
-
Immersion bioprinting of hyaluronan and collagen bioink-supported 3D patient-derived brain tumor organoids.Biomed Mater. 2022 Dec 2;18(1). doi: 10.1088/1748-605X/aca05d. Biomed Mater. 2022. PMID: 36332268
-
Challenges in Bio-fabrication of Organoid Cultures.Adv Exp Med Biol. 2018;1107:53-71. doi: 10.1007/5584_2018_216. Adv Exp Med Biol. 2018. PMID: 29855825 Review.
Cited by
-
Minimal-Invasive 3D Laser Printing of Microimplants in Organismo.Adv Sci (Weinh). 2024 Aug;11(30):e2401110. doi: 10.1002/advs.202401110. Epub 2024 Jun 12. Adv Sci (Weinh). 2024. PMID: 38864352 Free PMC article.
-
Recent advances on thermosensitive hydrogels-mediated precision therapy.Asian J Pharm Sci. 2024 Jun;19(3):100911. doi: 10.1016/j.ajps.2024.100911. Epub 2024 Apr 14. Asian J Pharm Sci. 2024. PMID: 38948400 Free PMC article. Review.
-
Special Issue: Bioceramics, Bioglasses, and Gels for Tissue Engineering.Gels. 2023 Jul 21;9(7):586. doi: 10.3390/gels9070586. Gels. 2023. PMID: 37504465 Free PMC article.
-
Designer cellular spheroids with DNA origami for drug screening.Sci Adv. 2024 Jul 19;10(29):eado9880. doi: 10.1126/sciadv.ado9880. Epub 2024 Jul 19. Sci Adv. 2024. PMID: 39028810 Free PMC article.
-
Bio-orthogonal tuning of matrix properties during 3D cell culture to induce morphological and phenotypic changes.Nat Protoc. 2024 Nov 5. doi: 10.1038/s41596-024-01066-z. Online ahead of print. Nat Protoc. 2024. PMID: 39501109 Review.
References
Publication types
MeSH terms
Substances
Grants and funding
LinkOut - more resources
Full Text Sources