(Patho)Physiology of Glycosylphosphatidylinositol-Anchored Proteins I: Localization at Plasma Membranes and Extracellular Compartments
- PMID: 37238725
- PMCID: PMC10216265
- DOI: 10.3390/biom13050855
(Patho)Physiology of Glycosylphosphatidylinositol-Anchored Proteins I: Localization at Plasma Membranes and Extracellular Compartments
Abstract
Glycosylphosphatidylinositol (GPI)-anchored proteins (APs) are anchored at the outer leaflet of plasma membranes (PMs) of all eukaryotic organisms studied so far by covalent linkage to a highly conserved glycolipid rather than a transmembrane domain. Since their first description, experimental data have been accumulating for the capability of GPI-APs to be released from PMs into the surrounding milieu. It became evident that this release results in distinct arrangements of GPI-APs which are compatible with the aqueous milieu upon loss of their GPI anchor by (proteolytic or lipolytic) cleavage or in the course of shielding of the full-length GPI anchor by incorporation into extracellular vesicles, lipoprotein-like particles and (lyso)phospholipid- and cholesterol-harboring micelle-like complexes or by association with GPI-binding proteins or/and other full-length GPI-APs. In mammalian organisms, the (patho)physiological roles of the released GPI-APs in the extracellular environment, such as blood and tissue cells, depend on the molecular mechanisms of their release as well as the cell types and tissues involved, and are controlled by their removal from circulation. This is accomplished by endocytic uptake by liver cells and/or degradation by GPI-specific phospholipase D in order to bypass potential unwanted effects of the released GPI-APs or their transfer from the releasing donor to acceptor cells (which will be reviewed in a forthcoming manuscript).
Keywords: (G)PI-specific phospholipase D (GPLD1); adipose cells; extracellular vesicles; glycosylphosphatidylinositol (GPI)-anchored proteins (GPI-APs); metabolic diseases; protein release; sulfonylurea drugs.
Conflict of interest statement
The authors declare no conflict of interest.
Figures
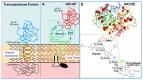
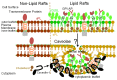
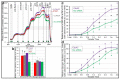
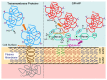
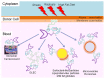
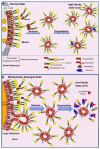
Similar articles
-
(Patho)Physiology of Glycosylphosphatidylinositol-Anchored Proteins II: Intercellular Transfer of Matter (Inheritance?) That Matters.Biomolecules. 2023 Jun 15;13(6):994. doi: 10.3390/biom13060994. Biomolecules. 2023. PMID: 37371574 Free PMC article. Review.
-
Transfer of Proteins from Cultured Human Adipose to Blood Cells and Induction of Anabolic Phenotype Are Controlled by Serum, Insulin and Sulfonylurea Drugs.Int J Mol Sci. 2023 Mar 2;24(5):4825. doi: 10.3390/ijms24054825. Int J Mol Sci. 2023. PMID: 36902257 Free PMC article.
-
The release of glycosylphosphatidylinositol-anchored proteins from the cell surface.Arch Biochem Biophys. 2018 Oct 15;656:1-18. doi: 10.1016/j.abb.2018.08.009. Epub 2018 Aug 16. Arch Biochem Biophys. 2018. PMID: 30120921 Review.
-
Age-dependent membrane release and degradation of full-length glycosylphosphatidylinositol-anchored proteins in rats.Mech Ageing Dev. 2020 Sep;190:111307. doi: 10.1016/j.mad.2020.111307. Epub 2020 Jul 3. Mech Ageing Dev. 2020. PMID: 32628941
-
Biological Role of the Intercellular Transfer of Glycosylphosphatidylinositol-Anchored Proteins: Stimulation of Lipid and Glycogen Synthesis.Int J Mol Sci. 2022 Jul 4;23(13):7418. doi: 10.3390/ijms23137418. Int J Mol Sci. 2022. PMID: 35806423 Free PMC article.
Cited by
-
Actin cytoskeleton differently regulates cell surface organization of GPI-anchored proteins in polarized epithelial cells and fibroblasts.Front Mol Biosci. 2024 May 7;11:1360142. doi: 10.3389/fmolb.2024.1360142. eCollection 2024. Front Mol Biosci. 2024. PMID: 38774234 Free PMC article.
-
(Patho)Physiology of Glycosylphosphatidylinositol-Anchored Proteins II: Intercellular Transfer of Matter (Inheritance?) That Matters.Biomolecules. 2023 Jun 15;13(6):994. doi: 10.3390/biom13060994. Biomolecules. 2023. PMID: 37371574 Free PMC article. Review.
-
Bone marrow stromal cell antigen 2: Tumor biology, signaling pathway and therapeutic targeting (Review).Oncol Rep. 2024 Mar;51(3):45. doi: 10.3892/or.2024.8704. Epub 2024 Jan 19. Oncol Rep. 2024. PMID: 38240088 Free PMC article. Review.
-
The Mechanism of Transcription Factor Swi6 in Regulating Growth and Pathogenicity of Ceratocystis fimbriata: Insights from Non-Targeted Metabolomics.Microorganisms. 2023 Oct 30;11(11):2666. doi: 10.3390/microorganisms11112666. Microorganisms. 2023. PMID: 38004677 Free PMC article.
-
A "poly-matter network" conception of biological inheritance.Genetica. 2024 Dec;152(4-6):211-230. doi: 10.1007/s10709-024-00216-1. Epub 2024 Oct 19. Genetica. 2024. PMID: 39425866 Free PMC article. Review.
References
Publication types
MeSH terms
Substances
Grants and funding
LinkOut - more resources
Full Text Sources
Research Materials
Miscellaneous