Label-Free Chemically and Molecularly Selective Magnetic Resonance Imaging
- PMID: 37235188
- PMCID: PMC10207347
- DOI: 10.1021/cbmi.3c00019
Label-Free Chemically and Molecularly Selective Magnetic Resonance Imaging
Abstract
Biomedical imaging, especially molecular imaging, has been a driving force in scientific discovery, technological innovation, and precision medicine in the past two decades. While substantial advances and discoveries in chemical biology have been made to develop molecular imaging probes and tracers, translating these exogenous agents to clinical application in precision medicine is a major challenge. Among the clinically accepted imaging modalities, magnetic resonance imaging (MRI) and magnetic resonance spectroscopy (MRS) exemplify the most effective and robust biomedical imaging tools. Both MRI and MRS enable a broad range of chemical, biological and clinical applications from determining molecular structures in biochemical analysis to imaging diagnosis and characterization of many diseases and image-guided interventions. Using chemical, biological, and nuclear magnetic resonance properties of specific endogenous metabolites and native MRI contrast-enhancing biomolecules, label-free molecular and cellular imaging with MRI can be achieved in biomedical research and clinical management of patients with various diseases. This review article outlines the chemical and biological bases of several label-free chemically and molecularly selective MRI and MRS methods that have been applied in imaging biomarker discovery, preclinical investigation, and image-guided clinical management. Examples are provided to demonstrate strategies for using endogenous probes to report the molecular, metabolic, physiological, and functional events and processes in living systems, including patients. Future perspectives on label-free molecular MRI and its challenges as well as potential solutions, including the use of rational design and engineered approaches to develop chemical and biological imaging probes to facilitate or combine with label-free molecular MRI, are discussed.
© 2023 The Authors. Co-published by Nanjing University and American Chemical Society.
Conflict of interest statement
The authors declare no competing financial interest.
Figures
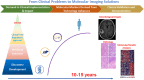
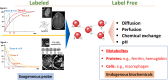
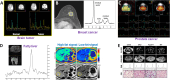
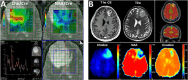
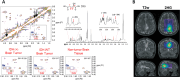
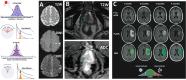
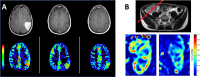
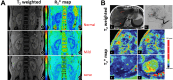
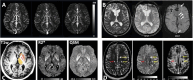
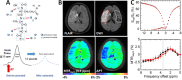
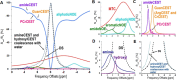
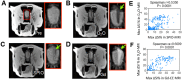
Similar articles
-
Translational Metabolomics of Head Injury: Exploring Dysfunctional Cerebral Metabolism with Ex Vivo NMR Spectroscopy-Based Metabolite Quantification.In: Kobeissy FH, editor. Brain Neurotrauma: Molecular, Neuropsychological, and Rehabilitation Aspects. Boca Raton (FL): CRC Press/Taylor & Francis; 2015. Chapter 25. In: Kobeissy FH, editor. Brain Neurotrauma: Molecular, Neuropsychological, and Rehabilitation Aspects. Boca Raton (FL): CRC Press/Taylor & Francis; 2015. Chapter 25. PMID: 26269925 Free Books & Documents. Review.
-
Hyperpolarized α-keto[1-13C]isocaproate as a 13C magnetic resonance spectroscopic agent for profiling branched chain amino acid metabolism in tumors.2010 Jan 15 [updated 2010 May 19]. In: Molecular Imaging and Contrast Agent Database (MICAD) [Internet]. Bethesda (MD): National Center for Biotechnology Information (US); 2004–2013. 2010 Jan 15 [updated 2010 May 19]. In: Molecular Imaging and Contrast Agent Database (MICAD) [Internet]. Bethesda (MD): National Center for Biotechnology Information (US); 2004–2013. PMID: 20641995 Free Books & Documents. Review.
-
Magnetic resonance imaging (MRI) for the assessment of myocardial viability: an evidence-based analysis.Ont Health Technol Assess Ser. 2010;10(15):1-45. Epub 2010 Jul 1. Ont Health Technol Assess Ser. 2010. PMID: 23074392 Free PMC article.
-
State-of-the-art accounts of hyperpolarized 15N-labeled molecular imaging probes for magnetic resonance spectroscopy and imaging.Chem Sci. 2022 May 17;13(25):7378-7391. doi: 10.1039/d2sc01264b. eCollection 2022 Jun 29. Chem Sci. 2022. PMID: 35872812 Free PMC article. Review.
-
Navigating Neural Landscapes: A Comprehensive Review of Magnetic Resonance Imaging (MRI) and Magnetic Resonance Spectroscopy (MRS) Applications in Epilepsy.Cureus. 2024 Mar 25;16(3):e56927. doi: 10.7759/cureus.56927. eCollection 2024 Mar. Cureus. 2024. PMID: 38665706 Free PMC article. Review.
Cited by
-
225Ac-Labeled Antibody for Fibroblast Activation Protein-Targeted Alpha Therapy.Chem Biomed Imaging. 2023 Aug 17;1(7):628-636. doi: 10.1021/cbmi.3c00067. eCollection 2023 Oct 23. Chem Biomed Imaging. 2023. PMID: 39474138 Free PMC article.
-
Activatable Multiplexed 19F NMR Probes for Dynamic Monitoring of Biomarkers Associated with Cellular Senescence.ACS Meas Sci Au. 2024 Aug 31;4(5):577-584. doi: 10.1021/acsmeasuresciau.4c00045. eCollection 2024 Oct 16. ACS Meas Sci Au. 2024. PMID: 39430968 Free PMC article.
References
Publication types
Grants and funding
LinkOut - more resources
Full Text Sources
Miscellaneous