The Isolation and In Vitro Differentiation of Primary Fetal Baboon Tracheal Epithelial Cells for the Study of SARS-CoV-2 Host-Virus Interactions
- PMID: 37112842
- PMCID: PMC10146425
- DOI: 10.3390/v15040862
The Isolation and In Vitro Differentiation of Primary Fetal Baboon Tracheal Epithelial Cells for the Study of SARS-CoV-2 Host-Virus Interactions
Abstract
The mucociliary airway epithelium lines the human airways and is the primary site of host-environmental interactions in the lung. Following virus infection, airway epithelial cells initiate an innate immune response to suppress virus replication. Therefore, defining the virus-host interactions of the mucociliary airway epithelium is critical for understanding the mechanisms that regulate virus infection, including Severe Acute Respiratory Syndrome Coronavirus 2 (SARS-CoV-2). Non-human primates (NHP) are closely related to humans and provide a model to study human disease. However, ethical considerations and high costs can restrict the use of in vivo NHP models. Therefore, there is a need to develop in vitro NHP models of human respiratory virus infection that would allow for rapidly characterizing virus tropism and the suitability of specific NHP species to model human infection. Using the olive baboon (Papio anubis), we have developed methodologies for the isolation, in vitro expansion, cryopreservation, and mucociliary differentiation of primary fetal baboon tracheal epithelial cells (FBTECs). Furthermore, we demonstrate that in vitro differentiated FBTECs are permissive to SARS-CoV-2 infection and produce a potent host innate-immune response. In summary, we have developed an in vitro NHP model that provides a platform for the study of SARS-CoV-2 infection and other human respiratory viruses.
Keywords: COVID-19; SARS-CoV-2; airway epithelium; air–liquid interface; baboon; immune response; inflammation; innate immunity; non-human primate.
Conflict of interest statement
All the authors declare they have no conflict of interest in relation to the subject matter or materials discussed in this manuscript. The funders had no role in study design, data collection, data analysis, decision to publish or preparation of the manuscript.
Figures
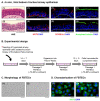
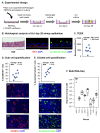
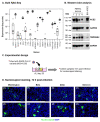
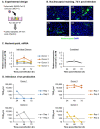
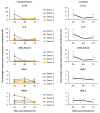
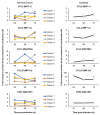
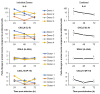
Similar articles
-
Long-Term Modeling of SARS-CoV-2 Infection of In Vitro Cultured Polarized Human Airway Epithelium.mBio. 2020 Nov 6;11(6):e02852-20. doi: 10.1128/mBio.02852-20. mBio. 2020. PMID: 33158999 Free PMC article.
-
SARS-CoV replication and pathogenesis in an in vitro model of the human conducting airway epithelium.Virus Res. 2008 Apr;133(1):33-44. doi: 10.1016/j.virusres.2007.03.013. Epub 2007 Apr 23. Virus Res. 2008. PMID: 17451829 Free PMC article. Review.
-
Type I and Type III Interferons Restrict SARS-CoV-2 Infection of Human Airway Epithelial Cultures.J Virol. 2020 Sep 15;94(19):e00985-20. doi: 10.1128/JVI.00985-20. Print 2020 Sep 15. J Virol. 2020. PMID: 32699094 Free PMC article.
-
Elevated temperature inhibits SARS-CoV-2 replication in respiratory epithelium independently of IFN-mediated innate immune defenses.PLoS Biol. 2021 Dec 21;19(12):e3001065. doi: 10.1371/journal.pbio.3001065. eCollection 2021 Dec. PLoS Biol. 2021. PMID: 34932557 Free PMC article.
-
Respiratory epithelial cell responses to SARS-CoV-2 in COVID-19.Thorax. 2022 Feb;77(2):203-209. doi: 10.1136/thoraxjnl-2021-217561. Epub 2021 Aug 17. Thorax. 2022. PMID: 34404754 Free PMC article. Review.
References
-
- Basil M.C., Katzen J., Engler A.E., Guo M., Herriges M.J., Kathiriya J.J., Windmueller R., Ysasi A.B., Zacharias W.J., Chapman H.A., et al. The Cellular and Physiological Basis for Lung Repair and Regeneration: Past, Present, and Future. Cell Stem. Cell. 2020;26:482–502. doi: 10.1016/j.stem.2020.03.009. - DOI - PMC - PubMed
-
- Hogan B.L., Barkauskas C.E., Chapman H.A., Epstein J.A., Jain R., Hsia C.C., Niklason L., Calle E., Le A., Randell S.H., et al. Repair and regeneration of the respiratory system: Complexity, plasticity, and mechanisms of lung stem cell function. Cell Stem. Cell. 2014;15:123–138. doi: 10.1016/j.stem.2014.07.012. - DOI - PMC - PubMed
Publication types
MeSH terms
Grants and funding
LinkOut - more resources
Full Text Sources
Medical
Molecular Biology Databases
Miscellaneous