Simulations of active zone structure and function at mammalian NMJs predict that loss of calcium channels alone is not sufficient to replicate LEMS effects
- PMID: 37073966
- PMCID: PMC10202491
- DOI: 10.1152/jn.00404.2022
Simulations of active zone structure and function at mammalian NMJs predict that loss of calcium channels alone is not sufficient to replicate LEMS effects
Abstract
Lambert-Eaton myasthenic syndrome (LEMS) is an autoimmune-mediated neuromuscular disease thought to be caused by autoantibodies against P/Q-type voltage-gated calcium channels (VGCCs), which attack and reduce the number of VGCCs within transmitter release sites (active zones; AZs) at the neuromuscular junction (NMJ), resulting in neuromuscular weakness. However, patients with LEMS also have antibodies to other neuronal proteins, and about 15% of patients with LEMS are seronegative for antibodies against VGCCs. We hypothesized that a reduction in the number of P/Q-type VGCCs alone is not sufficient to explain LEMS effects on transmitter release. Here, we used a computational model to study a variety of LEMS-mediated effects on AZ organization and transmitter release constrained by electron microscopic, pharmacological, immunohistochemical, voltage imaging, and electrophysiological observations. We show that models of healthy AZs can be modified to predict the transmitter release and short-term facilitation characteristics of LEMS and that in addition to a decrease in the number of AZ VGCCs, disruption in the organization of AZ proteins, a reduction in AZ number, a reduction in the amount of synaptotagmin, and the compensatory expression of L-type channels outside the remaining AZs are important contributors to LEMS-mediated effects on transmitter release. Furthermore, our models predict that antibody-mediated removal of synaptotagmin in combination with disruption in AZ organization alone could mimic LEMS effects without the removal of VGCCs (a seronegative model). Overall, our results suggest that LEMS pathophysiology may be caused by a collection of pathological alterations to AZs at the NMJ, rather than by a simple loss of VGCCs.NEW & NOTEWORTHY We used a computational model of the active zone (AZ) in the mammalian neuromuscular junction to investigate Lambert-Eaton myasthenic syndrome (LEMS) pathophysiology. This model suggests that disruptions in presynaptic active zone organization and protein content (particularly synaptotagmin), beyond the simple removal of presynaptic calcium channels, play an important role in LEMS pathophysiology.
Keywords: Lambert-Eaton myasthenic syndrome; MCell modeling; active zone; neuromuscular junction.
Conflict of interest statement
No conflicts of interest, financial or otherwise, are declared by the authors.
Figures
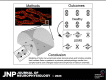
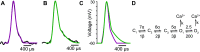
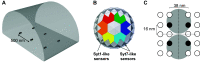
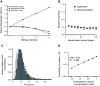
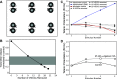
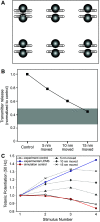
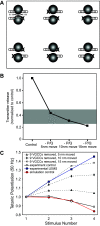
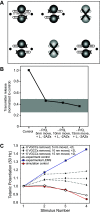
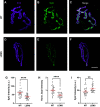
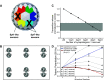
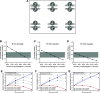
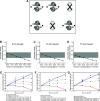
Similar articles
-
Neuromuscular Active Zone Structure and Function in Healthy and Lambert-Eaton Myasthenic Syndrome States.Biomolecules. 2022 May 24;12(6):740. doi: 10.3390/biom12060740. Biomolecules. 2022. PMID: 35740866 Free PMC article. Review.
-
Pathogenic autoantibodies in the lambert-eaton myasthenic syndrome.Ann N Y Acad Sci. 2003 Sep;998:187-95. doi: 10.1196/annals.1254.019. Ann N Y Acad Sci. 2003. PMID: 14592874
-
[P/Q-type Calcium Channel Antibodies in Lambert-Eaton Myasthenic Syndrome].Brain Nerve. 2018 Apr;70(4):341-355. doi: 10.11477/mf.1416201007. Brain Nerve. 2018. PMID: 29632282 Japanese.
-
Ca2+ channels as targets of neurological disease: Lambert-Eaton Syndrome and other Ca2+ channelopathies.J Bioenerg Biomembr. 2003 Dec;35(6):697-718. doi: 10.1023/b:jobb.0000008033.02320.10. J Bioenerg Biomembr. 2003. PMID: 15000529 Review.
-
Lambert-Eaton myasthenic syndrome: search for alternative autoimmune targets and possible compensatory mechanisms based on presynaptic calcium homeostasis.J Neuroimmunol. 2008 Sep 15;201-202:145-52. doi: 10.1016/j.jneuroim.2008.04.040. Epub 2008 Jul 24. J Neuroimmunol. 2008. PMID: 18653248 Review.
References
-
- Tarr TB, Malick W, Liang M, Valdomir G, Frasso M, Lacomis D, Reddel SW, Garcia-Ocano A, Wipf P, Meriney SD. Evaluation of a novel calcium channel agonist for therapeutic potential in Lambert-Eaton myasthenic syndrome. J Neurosci 33: 10559–10567, 2013. doi:10.1523/JNEUROSCI.4629-12.2013. - DOI - PMC - PubMed
Publication types
MeSH terms
Substances
LinkOut - more resources
Full Text Sources