Beyond Conventional Sensing: Hybrid Plasmonic Metasurfaces and Bound States in the Continuum
- PMID: 37049354
- PMCID: PMC10097206
- DOI: 10.3390/nano13071261
Beyond Conventional Sensing: Hybrid Plasmonic Metasurfaces and Bound States in the Continuum
Abstract
Fano resonances result from the strong coupling and interference between a broad background state and a narrow, almost discrete state, leading to the emergence of asymmetric scattering spectral profiles. Under certain conditions, Fano resonances can experience a collapse of their width due to the destructive interference of strongly coupled modes, resulting in the formation of bound states in the continuum (BIC). In such cases, the modes are simultaneously localized in the nanostructure and coexist with radiating waves, leading to an increase in the quality factor, which is virtually unlimited. In this work, we report on the design of a layered hybrid plasmonic-dielectric metasurface that facilitates strong mode coupling and the formation of BIC, resulting in resonances with a high quality factor. We demonstrate the possibility of controlling Fano resonances and tuning Rabi splitting using the nanoantenna dimensions. We also experimentally demonstrate the generalized Kerker effect in a binary arrangement of silicon nanodisks, which allows for the tuning of the collective modes and creates new photonic functionalities and improved sensing capabilities. Our findings have promising implications for developing plasmonic sensors that leverage strong light-matter interactions in hybrid metasurfaces.
Keywords: Fano resonances; Kerker effect; Rabi splitting; binary arrangement; light-matter interactions; nanostructure; plasmonic sensors; silicon nanodisks; strong coupling.
Conflict of interest statement
The authors declare no conflict of interest.
Figures
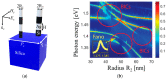
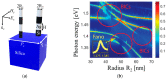
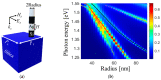
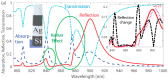
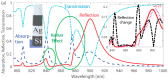
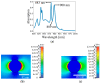
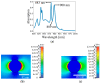
Similar articles
-
Bound States in the Continuum in Anisotropic Plasmonic Metasurfaces.Nano Lett. 2020 Sep 9;20(9):6351-6356. doi: 10.1021/acs.nanolett.0c01752. Epub 2020 Jun 10. Nano Lett. 2020. PMID: 32479094
-
Hybrid anisotropic plasmonic metasurfaces with multiple resonances of focused light beams.Nano Lett. 2021 Oct 27;21(20):8917-8923. doi: 10.1021/acs.nanolett.1c02751. Epub 2021 Aug 30. Nano Lett. 2021. PMID: 34459611
-
Observation of Fano resonances in all-dielectric nanoparticle oligomers.Small. 2014 May 28;10(10):1985-90. doi: 10.1002/smll.201303612. Epub 2014 Feb 25. Small. 2014. PMID: 24616191
-
Dielectric Resonance-Based Optical Metasurfaces: From Fundamentals to Applications.iScience. 2020 Nov 26;23(12):101868. doi: 10.1016/j.isci.2020.101868. eCollection 2020 Dec 18. iScience. 2020. PMID: 33319185 Free PMC article. Review.
-
Photonic Bound States in the Continuum in Nanostructures.Materials (Basel). 2023 Nov 10;16(22):7112. doi: 10.3390/ma16227112. Materials (Basel). 2023. PMID: 38005042 Free PMC article. Review.
Cited by
-
Resonant Metasurfaces with Van Der Waals Hyperbolic Nanoantennas and Extreme Light Confinement.Nanomaterials (Basel). 2024 Sep 23;14(18):1539. doi: 10.3390/nano14181539. Nanomaterials (Basel). 2024. PMID: 39330695 Free PMC article.
-
Optical Processes behind Plasmonic Applications.Nanomaterials (Basel). 2023 Apr 3;13(7):1270. doi: 10.3390/nano13071270. Nanomaterials (Basel). 2023. PMID: 37049363 Free PMC article. Review.
-
Recent advances in the metamaterial and metasurface-based biosensor in the gigahertz, terahertz, and optical frequency domains.Heliyon. 2024 Jun 21;10(13):e33272. doi: 10.1016/j.heliyon.2024.e33272. eCollection 2024 Jul 15. Heliyon. 2024. PMID: 39040247 Free PMC article. Review.
References
-
- Gallinet B., Martin O.J.F. Ab initio theory of Fano resonances in plasmonic nanostructures and metamaterials. Phys. Rev. B. 2011;83:235427. doi: 10.1103/PhysRevB.83.235427. - DOI
-
- Limonov M.F., Rybin M.V., Poddubny A.N., Kivshar Y.S. Fano resonances in photonics. Nat. Photonics. 2017;11:543–554. doi: 10.1038/nphoton.2017.142. - DOI
Grants and funding
LinkOut - more resources
Full Text Sources