Recent Advances in Biosensor Technologies for Point-of-Care Urinalysis
- PMID: 36421138
- PMCID: PMC9688579
- DOI: 10.3390/bios12111020
Recent Advances in Biosensor Technologies for Point-of-Care Urinalysis
Abstract
Human urine samples are non-invasive, readily available, and contain several components that can provide useful indicators of the health status of patients. Hence, urine is a desirable and important template to aid in the diagnosis of common clinical conditions. Conventional methods such as dipstick tests, urine culture, and urine microscopy are commonly used for urinalysis. Among them, the dipstick test is undoubtedly the most popular owing to its ease of use, low cost, and quick response. Despite these advantages, the dipstick test has limitations in terms of sensitivity, selectivity, reusability, and quantitative evaluation of diseases. Various biosensor technologies give it the potential for being developed into point-of-care (POC) applications by overcoming these limitations of the dipstick test. Here, we present a review of the biosensor technologies available to identify urine-based biomarkers that are typically detected by the dipstick test and discuss the present limitations and challenges that future development for their translation into POC applications for urinalysis.
Keywords: biosensors; clinical application for urinalysis; dipstick test; point-of-care urinalysis; urine sensor.
Conflict of interest statement
The authors declare no conflict of interest.
Figures
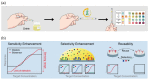
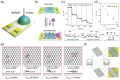
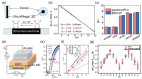
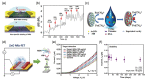
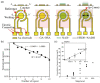
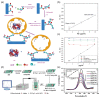
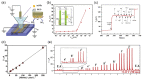
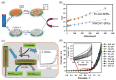
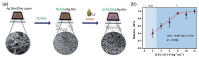
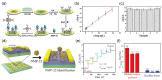
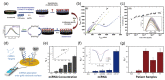
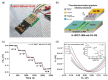
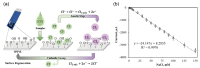
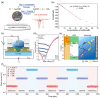
Similar articles
-
Comparison of test characteristics of urine dipstick and urinalysis at various test cutoff points.Ann Emerg Med. 2001 Nov;38(5):505-12. doi: 10.1067/mem.2001.119427. Ann Emerg Med. 2001. PMID: 11679861
-
Current and emerging trends in point-of-care urinalysis tests.Expert Rev Mol Diagn. 2020 Jan;20(1):69-84. doi: 10.1080/14737159.2020.1699063. Epub 2019 Dec 12. Expert Rev Mol Diagn. 2020. PMID: 31795785 Free PMC article. Review.
-
Dipstick screening for urinary tract infection in febrile infants.Pediatrics. 2014 May;133(5):e1121-7. doi: 10.1542/peds.2013-3291. Pediatrics. 2014. PMID: 24777232 Free PMC article.
-
Fast and Sensitive: Automated Point-of-Care Urine Dips.Pediatr Emerg Care. 2020 Oct;36(10):486-488. doi: 10.1097/PEC.0000000000001357. Pediatr Emerg Care. 2020. PMID: 29189595
-
Dipstick analysis of urine chemistry: benefits and limitations of dry chemistry-based assays.Postgrad Med. 2020 Apr;132(3):225-233. doi: 10.1080/00325481.2019.1679540. Epub 2019 Oct 19. Postgrad Med. 2020. PMID: 31609156 Review.
Cited by
-
Innovative Biosensor Technologies in the Diagnosis of Urinary Tract Infections: A Comprehensive Literature Review.Indian J Microbiol. 2024 Sep;64(3):894-909. doi: 10.1007/s12088-024-01359-7. Epub 2024 Aug 2. Indian J Microbiol. 2024. PMID: 39282176 Review.
-
A 3D-Printed Do-It-Yourself ELISA Plate Reader as a Biosensor Tested on TNFα Assay.Biosensors (Basel). 2024 Jul 6;14(7):331. doi: 10.3390/bios14070331. Biosensors (Basel). 2024. PMID: 39056607 Free PMC article.
-
Urine Test Strip Quantitative Assay with a Smartphone Camera.Int J Anal Chem. 2024 Mar 18;2024:6004970. doi: 10.1155/2024/6004970. eCollection 2024. Int J Anal Chem. 2024. PMID: 38529171 Free PMC article.
-
Advances in Fiber-Based Wearable Sensors for Personal Digital Health Monitoring.Materials (Basel). 2023 Nov 29;16(23):7428. doi: 10.3390/ma16237428. Materials (Basel). 2023. PMID: 38068172 Free PMC article. Review.
-
Urinary Biomarkers and Point-of-Care Urinalysis Devices for Early Diagnosis and Management of Disease: A Review.Biomedicines. 2023 Mar 29;11(4):1051. doi: 10.3390/biomedicines11041051. Biomedicines. 2023. PMID: 37189669 Free PMC article. Review.
References
-
- Fogazzi G.B. The Urinary Sediment. An Integrated View. Penerbit Buku Kompas; Trento, Italy: 2010.
Publication types
MeSH terms
Substances
Grants and funding
LinkOut - more resources
Full Text Sources
Medical