DGKα, Bridging Membrane Shape Changes with Specific Molecular Species of DAG/PA: Implications in Cancer and Immunosurveillance
- PMID: 36358678
- PMCID: PMC9655555
- DOI: 10.3390/cancers14215259
DGKα, Bridging Membrane Shape Changes with Specific Molecular Species of DAG/PA: Implications in Cancer and Immunosurveillance
Abstract
Cancer immunotherapy has revolutionized the oncology field. Despite the success, new molecular targets are needed to increase the percentage of patients that benefits from this therapy. Diacylglycerol kinase α (DGKα) has gathered great attention as a potential molecular target in immunotherapy because of its role in cancer proliferation and immunosuppression. DGKα catalyzes the ATP-dependent phosphorylation of diacylglycerol (DAG) to produce phosphatidic acid (PA). Since both lipids are potent signaling messengers, DGKα acts as a switch between different signaling pathways. Its role in cancer and immunosuppression has long been ascribed to the regulation of DAG/PA levels. However, this paradigm has been challenged with the identification of DGKα substrate acyl chain specificity, which suggests its role in signaling could be specific to DAG/PA molecular species. In several biological processes where DGKα plays a role, large membrane morphological changes take place. DGKα substrate specificity depends on the shape of the membrane that the enzyme binds to. Hence, DGKα can act as a bridge between large membrane morphological changes and the regulation of specific molecular species of DAG/PA. Bearing in mind the potential therapeutic benefits of targeting DGKα, here, the role of DGKα in cancer and T cell biology with a focus on the modulation of its enzymatic properties by membrane shape is reviewed. The goal is to contribute to a global understanding of the molecular mechanisms governing DGKα biology. This will pave the way for future experimentation and, consequently, the design of better, more potent therapeutic strategies aiming at improving the health outcomes of cancer patients.
Keywords: cancer; cellular signaling; diacylglycerol; diacylglycerol kinase α; immune surveillance; immunotherapy; phosphatidic acid; regulation of enzymes by membrane shape; substrate acyl chain specificity.
Conflict of interest statement
The authors declare no conflict of interest.
Figures
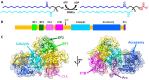
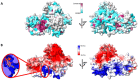
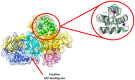
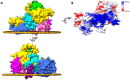
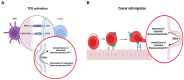
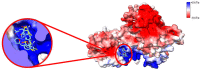
Similar articles
-
Membrane morphology determines diacylglycerol kinase α substrate acyl chain specificity.FASEB J. 2021 Jun;35(6):e21602. doi: 10.1096/fj.202100264R. FASEB J. 2021. PMID: 33977628
-
Molecular Pathways: Targeting Diacylglycerol Kinase Alpha in Cancer.Clin Cancer Res. 2015 Nov 15;21(22):5008-12. doi: 10.1158/1078-0432.CCR-15-0413. Epub 2015 Sep 29. Clin Cancer Res. 2015. PMID: 26420856 Free PMC article.
-
The ζ isoform of diacylglycerol kinase plays a predominant role in regulatory T cell development and TCR-mediated ras signaling.Sci Signal. 2013 Nov 26;6(303):ra102. doi: 10.1126/scisignal.2004373. Sci Signal. 2013. PMID: 24280043 Free PMC article.
-
Diacylglycerol Kinases: Shaping Diacylglycerol and Phosphatidic Acid Gradients to Control Cell Polarity.Front Cell Dev Biol. 2016 Nov 29;4:140. doi: 10.3389/fcell.2016.00140. eCollection 2016. Front Cell Dev Biol. 2016. PMID: 27965956 Free PMC article. Review.
-
The Roles of Diacylglycerol Kinase α in Cancer Cell Proliferation and Apoptosis.Cancers (Basel). 2021 Oct 16;13(20):5190. doi: 10.3390/cancers13205190. Cancers (Basel). 2021. PMID: 34680338 Free PMC article. Review.
Cited by
-
Phospholipases and Membrane Curvature: What Is Happening at the Surface?Membranes (Basel). 2023 Feb 3;13(2):190. doi: 10.3390/membranes13020190. Membranes (Basel). 2023. PMID: 36837693 Free PMC article. Review.
-
Lipid phosphorylation by a diacylglycerol kinase suppresses ABA biosynthesis to regulate plant stress responses.Mol Plant. 2024 Feb 5;17(2):342-358. doi: 10.1016/j.molp.2024.01.003. Epub 2024 Jan 19. Mol Plant. 2024. PMID: 38243594 Free PMC article.
References
Publication types
Grants and funding
LinkOut - more resources
Full Text Sources