Zinc finger structure determination by NMR: Why zinc fingers can be a handful
- PMID: 36113918
- PMCID: PMC7614390
- DOI: 10.1016/j.pnmrs.2022.07.001
Zinc finger structure determination by NMR: Why zinc fingers can be a handful
Abstract
Zinc fingers can be loosely defined as protein domains containing one or more tetrahedrally-co-ordinated zinc ions whose role is to stabilise the structure rather than to be involved in enzymatic chemistry; such zinc ions are often referred to as "structural zincs". Although structural zincs can occur in proteins of any size, they assume particular significance for very small protein domains, where they are often essential for maintaining a folded state. Such small structures, that sometimes have only marginal stability, can present particular difficulties in terms of sample preparation, handling and structure determination, and early on they gained a reputation for being resistant to crystallisation. As a result, NMR has played a more prominent role in structural studies of zinc finger proteins than it has for many other types of proteins. This review will present an overview of the particular issues that arise for structure determination of zinc fingers by NMR, and ways in which these may be addressed.
Keywords: Metallothionein; NMR spectroscopy; NMR structure determination; Protein structure; Zinc finger.
Copyright © 2022 MRC Laboratory of Molecular Biology. Published by Elsevier B.V. All rights reserved.
Conflict of interest statement
Declaration of Competing Interest The authors declare the following financial interests/personal relationships which may be considered as potential competing interests: I am the Co-ordinating Editor for the journal to which I am submitting. The article will be handled exclusively by other members of the Editorial Board.
Figures
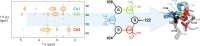
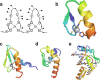
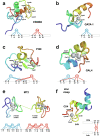
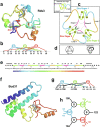
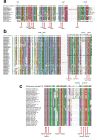
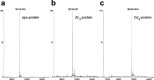
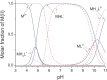
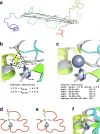
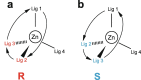
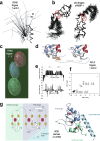
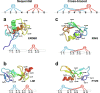
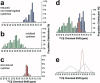
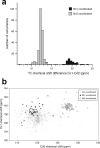
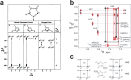
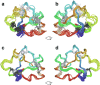
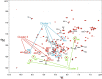
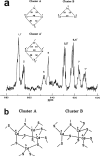
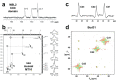
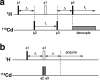
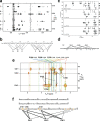
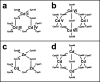
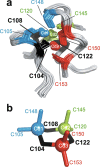
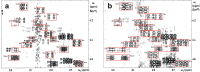
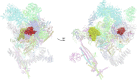
Similar articles
-
1H NMR structure and biological studies of the His23-->Cys mutant nucleocapsid protein of HIV-1 indicate that the conformation of the first zinc finger is critical for virus infectivity.Biochemistry. 1994 Oct 4;33(39):11707-16. doi: 10.1021/bi00205a006. Biochemistry. 1994. PMID: 7918387
-
Protein motifs 5. Zinc fingers.FASEB J. 1995 May;9(8):597-604. FASEB J. 1995. PMID: 7768350
-
The six zinc fingers of metal-responsive element binding transcription factor-1 form stable and quasi-ordered structures with relatively small differences in zinc affinities.J Biol Chem. 2005 Aug 5;280(31):28529-40. doi: 10.1074/jbc.M505217200. Epub 2005 May 31. J Biol Chem. 2005. PMID: 16055450
-
Zinc finger motif for single-stranded nucleic acids? Investigations by nuclear magnetic resonance.J Cell Biochem. 1991 Jan;45(1):41-8. doi: 10.1002/jcb.240450110. J Cell Biochem. 1991. PMID: 2005183 Review.
-
Zinc finger domains as therapeutic targets for metal-based compounds - an update.Metallomics. 2019 Jan 23;11(1):15-28. doi: 10.1039/c8mt00262b. Metallomics. 2019. PMID: 30303505 Review.
Cited by
-
Mode of SUV420H2 heterochromatin localization through multiple HP1 binding motifs in the heterochromatic targeting module.Genes Cells. 2024 May;29(5):361-379. doi: 10.1111/gtc.13109. Epub 2024 Feb 25. Genes Cells. 2024. PMID: 38403935 Free PMC article.
-
Zinc in Prostate Health and Disease: A Mini Review.Biomedicines. 2022 Dec 10;10(12):3206. doi: 10.3390/biomedicines10123206. Biomedicines. 2022. PMID: 36551962 Free PMC article. Review.
-
Structure and RNA-binding of the helically extended Roquin CCCH-type zinc finger.Nucleic Acids Res. 2024 Sep 9;52(16):9838-9853. doi: 10.1093/nar/gkae555. Nucleic Acids Res. 2024. PMID: 38953172 Free PMC article.
-
Zinc Deficiency as a General Feature of Cancer: a Review of the Literature.Biol Trace Elem Res. 2024 May;202(5):1937-1947. doi: 10.1007/s12011-023-03818-6. Epub 2023 Sep 2. Biol Trace Elem Res. 2024. PMID: 37658952 Free PMC article. Review.
-
Predicted Membrane-Associated Domains in Proteins Encoded by Novel Monopartite Plant RNA Viruses Related to Members of the Family Benyviridae.Int J Mol Sci. 2023 Jul 29;24(15):12161. doi: 10.3390/ijms241512161. Int J Mol Sci. 2023. PMID: 37569537 Free PMC article.
References
-
- Lee M.S., Gippert G.P., Soman K.V., Case D.A., Wright P.E. Three-dimensional solution structure of a single zinc finger DNA-binding domain. Science. 1989;245:635–637. - PubMed
-
- Parraga G., Horvath S.J., Eisen A., Taylor W.E., Hood L., Young E.T., Klevit R.E. Zinc-dependent structure of a single-finger domain of yeast ADR1. Science. 1988;241:1489–1492. - PubMed
-
- Klevit R.E., Herriott J.R., Horvath S.J. Solution structure of a zinc finger domain of yeast ADR1. Proteins. 1990;7:215–226. - PubMed
Publication types
MeSH terms
Substances
Grants and funding
LinkOut - more resources
Full Text Sources
Research Materials