In vivo characterization of Drosophila golgins reveals redundancy and plasticity of vesicle capture at the Golgi apparatus
- PMID: 36103876
- PMCID: PMC9849145
- DOI: 10.1016/j.cub.2022.08.054
In vivo characterization of Drosophila golgins reveals redundancy and plasticity of vesicle capture at the Golgi apparatus
Abstract
The Golgi is the central sorting station in the secretory pathway and thus the destination of transport vesicles arriving from the endoplasmic reticulum and endosomes and from within the Golgi itself. Cell viability, therefore, requires that the Golgi accurately receives multiple classes of vesicle. One set of proteins proposed to direct vesicle arrival at the Golgi are the golgins, long coiled-coil proteins localized to specific parts of the Golgi stack. In mammalian cells, three of the golgins, TMF, golgin-84, and GMAP-210, can capture intra-Golgi transport vesicles when placed in an ectopic location. However, the individual golgins are not required for cell viability, and mouse knockout mutants only have defects in specific tissues. To further illuminate this system, we examine the Drosophila orthologs of these three intra-Golgi golgins. We show that ectopic forms can capture intra-Golgi transport vesicles, but strikingly, the cargo present in the vesicles captured by each golgin varies between tissues. Loss-of-function mutants show that the golgins are individually dispensable, although the loss of TMF recapitulates the male fertility defects observed in mice. However, the deletion of multiple golgins results in defects in glycosylation and loss of viability. Examining the vesicles captured by a particular golgin when another golgin is missing reveals that the vesicle content in one tissue changes to resemble that of a different tissue. This reveals a plasticity in Golgi organization between tissues, providing an explanation for why the Golgi is sufficiently robust to tolerate the loss of many of the individual components of its membrane traffic machinery.
Keywords: Drosophila melanogaster; Golgi apparatus; ntra-Golgi transport; vesicle tether.
Copyright © 2022 The Author(s). Published by Elsevier Inc. All rights reserved.
Conflict of interest statement
Declaration of interests S.M. is a member of the Advisory Board of Current Biology. The authors declare no competing interests.
Figures
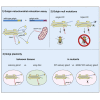
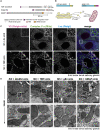
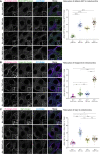
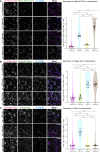
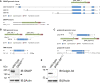
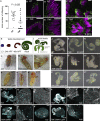
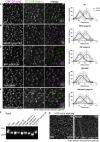
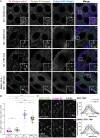
Similar articles
-
The golgin coiled-coil proteins capture different types of transport carriers via distinct N-terminal motifs.BMC Biol. 2017 Jan 26;15(1):3. doi: 10.1186/s12915-016-0345-3. BMC Biol. 2017. PMID: 28122620 Free PMC article.
-
Membrane trafficking. The specificity of vesicle traffic to the Golgi is encoded in the golgin coiled-coil proteins.Science. 2014 Oct 31;346(6209):1256898. doi: 10.1126/science.1256898. Science. 2014. PMID: 25359980 Free PMC article.
-
Finding the Golgi: Golgin Coiled-Coil Proteins Show the Way.Trends Cell Biol. 2016 Jun;26(6):399-408. doi: 10.1016/j.tcb.2016.02.005. Epub 2016 Mar 11. Trends Cell Biol. 2016. PMID: 26972448 Review.
-
Living on the edge: the role of Atgolgin-84A at the plant ER-Golgi interface.J Microsc. 2020 Nov;280(2):158-173. doi: 10.1111/jmi.12946. Epub 2020 Jul 31. J Microsc. 2020. PMID: 32700322
-
The Physiological Functions of the Golgin Vesicle Tethering Proteins.Front Cell Dev Biol. 2019 Jun 18;7:94. doi: 10.3389/fcell.2019.00094. eCollection 2019. Front Cell Dev Biol. 2019. PMID: 31316978 Free PMC article. Review.
Cited by
-
p24-Tango1 interactions ensure ER-Golgi interface stability and efficient transport.J Cell Biol. 2024 May 6;223(5):e202309045. doi: 10.1083/jcb.202309045. Epub 2024 Mar 12. J Cell Biol. 2024. PMID: 38470362 Free PMC article.
-
The FAM114A proteins are adaptors for the recycling of Golgi enzymes.J Cell Sci. 2024 Sep 1;137(17):jcs262160. doi: 10.1242/jcs.262160. Epub 2024 Sep 6. J Cell Sci. 2024. PMID: 39129673 Free PMC article.
-
Loss of dihydroceramide desaturase drives neurodegeneration by disrupting endoplasmic reticulum and lipid droplet homeostasis in glial cells.bioRxiv [Preprint]. 2024 May 21:2024.01.01.573836. doi: 10.1101/2024.01.01.573836. bioRxiv. 2024. PMID: 38260379 Free PMC article. Preprint.
References
Publication types
MeSH terms
Substances
Grants and funding
LinkOut - more resources
Full Text Sources
Molecular Biology Databases