Screening Biophysical Sensors and Neurite Outgrowth Actuators in Human Induced-Pluripotent-Stem-Cell-Derived Neurons
- PMID: 36010547
- PMCID: PMC9406775
- DOI: 10.3390/cells11162470
Screening Biophysical Sensors and Neurite Outgrowth Actuators in Human Induced-Pluripotent-Stem-Cell-Derived Neurons
Abstract
All living cells maintain a charge distribution across their cell membrane (membrane potential) by carefully controlled ion fluxes. These bioelectric signals regulate cell behavior (such as migration, proliferation, differentiation) as well as higher-level tissue and organ patterning. Thus, voltage gradients represent an important parameter for diagnostics as well as a promising target for therapeutic interventions in birth defects, injury, and cancer. However, despite much progress in cell and molecular biology, little is known about bioelectric states in human stem cells. Here, we present simple methods to simultaneously track ion dynamics, membrane voltage, cell morphology, and cell activity (pH and ROS), using fluorescent reporter dyes in living human neurons derived from induced neural stem cells (hiNSC). We developed and tested functional protocols for manipulating ion fluxes, membrane potential, and cell activity, and tracking neural responses to injury and reinnervation in vitro. Finally, using morphology sensor, we tested and quantified the ability of physiological actuators (neurotransmitters and pH) to manipulate nerve repair and reinnervation. These methods are not specific to a particular cell type and should be broadly applicable to the study of bioelectrical controls across a wide range of combinations of models and endpoints.
Keywords: GABA; acetylcholine; bioelectricity; hiNSC; ion flux; live sensor dyes; membrane potential; pH; serotonin.
Conflict of interest statement
The authors declare no competing financial interests.
Figures
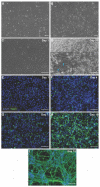
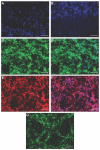
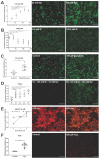
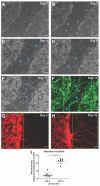
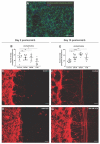
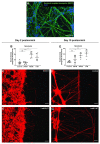
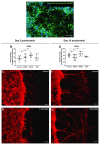
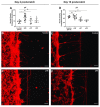
Similar articles
-
Neurite outgrowth in human induced pluripotent stem cell-derived neurons as a high-throughput screen for developmental neurotoxicity or neurotoxicity.Neurotoxicology. 2016 Mar;53:271-281. doi: 10.1016/j.neuro.2016.02.003. Epub 2016 Feb 4. Neurotoxicology. 2016. PMID: 26854185
-
Human Induced Pluripotent Stem Cell-Derived Neural Cells from Alzheimer's Disease Patients Exhibited Different Susceptibility to Oxidative Stress.Stem Cells Dev. 2020 Nov 15;29(22):1444-1456. doi: 10.1089/scd.2020.0103. Epub 2020 Oct 29. Stem Cells Dev. 2020. PMID: 32988331
-
An Electrophysiological and Pharmacological Study of the Properties of Human iPSC-Derived Neurons for Drug Discovery.Cells. 2021 Jul 31;10(8):1953. doi: 10.3390/cells10081953. Cells. 2021. PMID: 34440722 Free PMC article.
-
Transcriptional regulation and specification of neural stem cells.Adv Exp Med Biol. 2013;786:129-55. doi: 10.1007/978-94-007-6621-1_8. Adv Exp Med Biol. 2013. PMID: 23696355 Review.
-
Large-scale biophysics: ion flows and regeneration.Trends Cell Biol. 2007 Jun;17(6):261-70. doi: 10.1016/j.tcb.2007.04.007. Epub 2007 May 10. Trends Cell Biol. 2007. PMID: 17498955 Review.
Cited by
-
Neurogenesis manifestations of solid tumor and tracer imaging studies: a narrative review.Am J Cancer Res. 2023 Mar 15;13(3):713-726. eCollection 2023. Am J Cancer Res. 2023. PMID: 37034231 Free PMC article. Review.
-
Advances in high throughput cell culture technologies for therapeutic screening and biological discovery applications.Bioeng Transl Med. 2023 Dec 4;9(3):e10627. doi: 10.1002/btm2.10627. eCollection 2024 May. Bioeng Transl Med. 2023. PMID: 38818120 Free PMC article. Review.
References
Publication types
MeSH terms
Grants and funding
LinkOut - more resources
Full Text Sources