Label-free three-photon imaging of intact human cerebral organoids for tracking early events in brain development and deficits in Rett syndrome
- PMID: 35904330
- PMCID: PMC9337854
- DOI: 10.7554/eLife.78079
Label-free three-photon imaging of intact human cerebral organoids for tracking early events in brain development and deficits in Rett syndrome
Abstract
Human cerebral organoids are unique in their development of progenitor-rich zones akin to ventricular zones from which neuronal progenitors differentiate and migrate radially. Analyses of cerebral organoids thus far have been performed in sectioned tissue or in superficial layers due to their high scattering properties. Here, we demonstrate label-free three-photon imaging of whole, uncleared intact organoids (~2 mm depth) to assess early events of early human brain development. Optimizing a custom-made three-photon microscope to image intact cerebral organoids generated from Rett Syndrome patients, we show defects in the ventricular zone volumetric structure of mutant organoids compared to isogenic control organoids. Long-term imaging live organoids reveals that shorter migration distances and slower migration speeds of mutant radially migrating neurons are associated with more tortuous trajectories. Our label-free imaging system constitutes a particularly useful platform for tracking normal and abnormal development in individual organoids, as well as for screening therapeutic molecules via intact organoid imaging.
Keywords: cerebral organoids; deep tissue imaging; label-free imaging; multiphoton imaging; neuroscience; none; physics of living systems; rett syndrome.
© 2022, Yildirim et al.
Conflict of interest statement
MY, CD, DF, VP, SC, JI, AN, LT, GM, PS, MS No competing interests declared
Figures
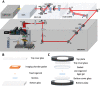
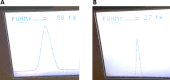
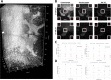
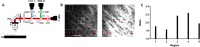
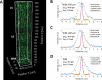
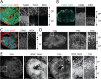
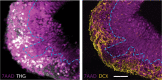
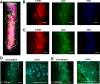
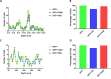
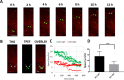
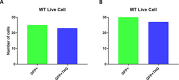
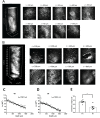
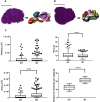
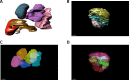
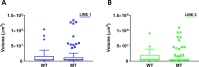
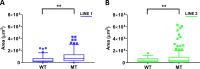
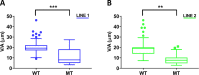
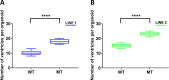
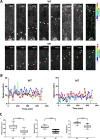
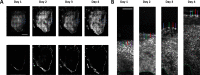
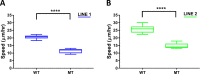
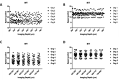
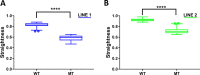
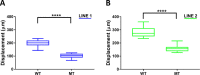
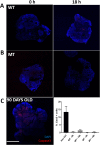
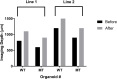
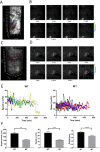
Similar articles
-
Applications of multiphoton microscopy in imaging cerebral and retinal organoids.Front Neurosci. 2024 Mar 5;18:1360482. doi: 10.3389/fnins.2024.1360482. eCollection 2024. Front Neurosci. 2024. PMID: 38505776 Free PMC article. Review.
-
KW-2449 and VPA exert therapeutic effects on human neurons and cerebral organoids derived from MECP2-null hESCs.Stem Cell Res Ther. 2022 Dec 27;13(1):534. doi: 10.1186/s13287-022-03216-0. Stem Cell Res Ther. 2022. PMID: 36575558 Free PMC article.
-
Modeling Rett Syndrome With Human Patient-Specific Forebrain Organoids.Front Cell Dev Biol. 2020 Dec 10;8:610427. doi: 10.3389/fcell.2020.610427. eCollection 2020. Front Cell Dev Biol. 2020. PMID: 33363173 Free PMC article.
-
A Simple Method for Generating Cerebral Organoids from Human Pluripotent Stem Cells.Int J Stem Cells. 2022 Feb 28;15(1):95-103. doi: 10.15283/ijsc21195. Int J Stem Cells. 2022. PMID: 35220295 Free PMC article.
-
Cerebral organoids exhibit mature neurons and astrocytes and recapitulate electrophysiological activity of the human brain.Neural Regen Res. 2019 May;14(5):757-761. doi: 10.4103/1673-5374.249283. Neural Regen Res. 2019. PMID: 30688257 Free PMC article. Review.
Cited by
-
Genetics of human brain development.Nat Rev Genet. 2024 Jan;25(1):26-45. doi: 10.1038/s41576-023-00626-5. Epub 2023 Jul 28. Nat Rev Genet. 2024. PMID: 37507490 Free PMC article. Review.
-
Involvement of extracellular vesicle microRNA clusters in developing healthy and Rett syndrome brain organoids.Cell Mol Life Sci. 2024 Sep 21;81(1):410. doi: 10.1007/s00018-024-05409-7. Cell Mol Life Sci. 2024. PMID: 39305343 Free PMC article.
-
Long-working-distance high-collection-efficiency three-photon microscopy for in vivo long-term imaging of zebrafish and organoids.iScience. 2024 Jul 19;27(8):110554. doi: 10.1016/j.isci.2024.110554. eCollection 2024 Aug 16. iScience. 2024. PMID: 39184441 Free PMC article.
-
Multiscale engineering of brain organoids for disease modeling.Adv Drug Deliv Rev. 2024 Jul;210:115344. doi: 10.1016/j.addr.2024.115344. Epub 2024 May 27. Adv Drug Deliv Rev. 2024. PMID: 38810702 Review.
-
Mitochondrial dysfunction and increased reactive oxygen species production in MECP2 mutant astrocytes and their impact on neurons.Sci Rep. 2024 Sep 4;14(1):20565. doi: 10.1038/s41598-024-71040-y. Sci Rep. 2024. PMID: 39232000 Free PMC article.
References
Publication types
MeSH terms
Associated data
Grants and funding
LinkOut - more resources
Full Text Sources
Medical
Research Materials