Efficient Correction of Oncogenic KRAS and TP53 Mutations through CRISPR Base Editing
- PMID: 35802645
- PMCID: PMC9437569
- DOI: 10.1158/0008-5472.CAN-21-2519
Efficient Correction of Oncogenic KRAS and TP53 Mutations through CRISPR Base Editing
Abstract
KRAS is the most frequently mutated oncogene in human cancer, and its activating mutations represent long-sought therapeutic targets. Programmable nucleases, particularly the CRISPR-Cas9 system, provide an attractive tool for genetically targeting KRAS mutations in cancer cells. Here, we show that cleavage of a panel of KRAS driver mutations suppresses growth in various human cancer cell lines, revealing their dependence on mutant KRAS. However, analysis of the remaining cell population after long-term Cas9 expression unmasked the occurence of oncogenic KRAS escape variants that were resistant to Cas9-cleavage. In contrast, the use of an adenine base editor to correct oncogenic KRAS mutations progressively depleted the targeted cells without the appearance of escape variants and allowed efficient and simultaneous correction of a cancer-associated TP53 mutation. Oncogenic KRAS and TP53 base editing was possible in patient-derived cancer organoids, suggesting that base editor approaches to correct oncogenic mutations could be developed for functional interrogation of vulnerabilities in a personalized manner for future precision oncology applications.
Significance: Repairing KRAS mutations with base editors can be used for providing a better understanding of RAS biology and may lay the foundation for improved treatments for KRAS-mutant cancers.
©2022 The Authors; Published by the American Association for Cancer Research.
Figures
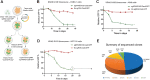
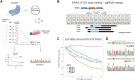
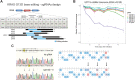
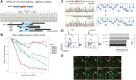
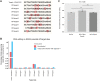
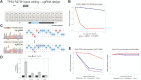
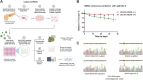
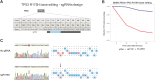
Similar articles
-
Selective targeting of the oncogenic KRAS G12S mutant allele by CRISPR/Cas9 induces efficient tumor regression.Theranostics. 2020 Apr 6;10(11):5137-5153. doi: 10.7150/thno.42325. eCollection 2020. Theranostics. 2020. PMID: 32308773 Free PMC article.
-
Targeting mutant KRAS with CRISPR-Cas9 controls tumor growth.Genome Res. 2018 Mar 1;28(3):374-382. doi: 10.1101/gr.223891.117. Genome Res. 2018. PMID: 29326299 Free PMC article.
-
Selective targeting of KRAS oncogenic alleles by CRISPR/Cas9 inhibits proliferation of cancer cells.Sci Rep. 2018 Aug 8;8(1):11879. doi: 10.1038/s41598-018-30205-2. Sci Rep. 2018. PMID: 30089886 Free PMC article.
-
Therapeutic Editing of the TP53 Gene: Is CRISPR/Cas9 an Option?Genes (Basel). 2020 Jun 25;11(6):704. doi: 10.3390/genes11060704. Genes (Basel). 2020. PMID: 32630614 Free PMC article. Review.
-
CRISPR and KRAS: a match yet to be made.J Biomed Sci. 2021 Nov 15;28(1):77. doi: 10.1186/s12929-021-00772-0. J Biomed Sci. 2021. PMID: 34781949 Free PMC article. Review.
Cited by
-
Translating p53-based therapies for cancer into the clinic.Nat Rev Cancer. 2024 Mar;24(3):192-215. doi: 10.1038/s41568-023-00658-3. Epub 2024 Jan 29. Nat Rev Cancer. 2024. PMID: 38287107 Review.
-
CRISPR prime editing for unconstrained correction of oncogenic KRAS variants.Commun Biol. 2023 Jun 30;6(1):681. doi: 10.1038/s42003-023-05052-1. Commun Biol. 2023. PMID: 37391511 Free PMC article.
-
Translation into Clinical Practice of the G1-G7 Molecular Subgroup Classification of Glioblastoma: Comprehensive Demographic and Molecular Pathway Profiling.Cancers (Basel). 2024 Jan 15;16(2):361. doi: 10.3390/cancers16020361. Cancers (Basel). 2024. PMID: 38254850 Free PMC article.
-
Nucleic Acid-Based Approaches to Tackle KRAS Mutant Cancers.Int J Mol Sci. 2023 Nov 29;24(23):16933. doi: 10.3390/ijms242316933. Int J Mol Sci. 2023. PMID: 38069255 Free PMC article. Review.
-
Targeting Mutant p53 for Cancer Treatment: Moving Closer to Clinical Use?Cancers (Basel). 2022 Sep 16;14(18):4499. doi: 10.3390/cancers14184499. Cancers (Basel). 2022. PMID: 36139658 Free PMC article. Review.
References
Publication types
MeSH terms
Substances
LinkOut - more resources
Full Text Sources
Research Materials
Miscellaneous