Improving in situ acoustic intensity estimates using MR acoustic radiation force imaging in combination with multifrequency MR elastography
- PMID: 35762849
- PMCID: PMC9439407
- DOI: 10.1002/mrm.29309
Improving in situ acoustic intensity estimates using MR acoustic radiation force imaging in combination with multifrequency MR elastography
Abstract
Purpose: Magnetic resonance acoustic radiation force imaging (MR-ARFI) enables focal spot localization during nonablative transcranial ultrasound therapies. As the acoustic radiation force is proportional to the applied acoustic intensity, measured MR-ARFI displacements could potentially be used to estimate the acoustic intensity at the target. However, variable brain stiffness is an obstacle. The goal of this study was to develop and assess a method to accurately estimate the acoustic intensity at the focus using MR-ARFI displacements in combination with viscoelastic properties obtained with multifrequency MR elastography (MRE).
Methods: Phantoms with a range of viscoelastic properties were fabricated, and MR-ARFI displacements were acquired within each phantom using multiple acoustic intensities. Voigt model parameters were estimated for each phantom based on storage and loss moduli measured using multifrequency MRE, and these were used to predict the relationship between acoustic intensity and measured displacement.
Results: Using assumed viscoelastic properties, MR-ARFI displacements alone could not accurately estimate acoustic intensity across phantoms. For example, acoustic intensities were underestimated in phantoms stiffer than the assumed stiffness and overestimated in phantoms softer than the assumed stiffness. This error was greatly reduced using individualized viscoelasticity measurements obtained from MRE.
Conclusion: We demonstrated that viscoelasticity information from MRE could be used in combination with MR-ARFI displacements to obtain more accurate estimates of acoustic intensity. Additionally, Voigt model viscosity parameters were found to be predictive of the relaxation rate of each phantom's time-varying displacement response, which could be used to optimize patient-specific MR-ARFI pulse sequences.
Keywords: MR-ARFI; MRgFUS; acoustic radiation force imaging; elastography; transcranial focused ultrasound.
© 2022 International Society for Magnetic Resonance in Medicine.
Figures
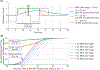
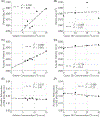
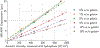
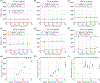
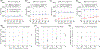
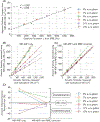
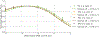
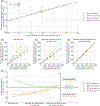
Similar articles
-
A simulation technique for 3D MR-guided acoustic radiation force imaging.Med Phys. 2015 Feb;42(2):674-84. doi: 10.1118/1.4905040. Med Phys. 2015. PMID: 25652481 Free PMC article.
-
Magnetic Resonance Acoustic Radiation Force Imaging (MR-ARFI) for the monitoring of High Intensity Focused Ultrasound (HIFU) ablation in anisotropic tissue.MAGMA. 2023 Oct;36(5):737-747. doi: 10.1007/s10334-023-01062-6. Epub 2023 Feb 1. MAGMA. 2023. PMID: 36723689
-
Ultrasound focusing using magnetic resonance acoustic radiation force imaging: application to ultrasound transcranial therapy.Med Phys. 2010 Jun;37(6):2934-42. doi: 10.1118/1.3395553. Med Phys. 2010. PMID: 20632605
-
Evaluation of the Elasticity of the Pancreas Using Acoustic Radiation Force Impulse Elastography in Patients with Acute Pancreatitis: A Systematic Review and Meta-Analysis.Ultrasound Med Biol. 2022 Mar;48(3):406-413. doi: 10.1016/j.ultrasmedbio.2021.11.013. Epub 2021 Dec 31. Ultrasound Med Biol. 2022. PMID: 34980499 Review.
-
Acoustic radiation force impulse of the liver.World J Gastroenterol. 2013 Aug 14;19(30):4841-9. doi: 10.3748/wjg.v19.i30.4841. World J Gastroenterol. 2013. PMID: 23946588 Free PMC article. Review.
Cited by
-
ITRUSST Consensus on Standardised Reporting for Transcranial Ultrasound Stimulation.ArXiv [Preprint]. 2024 Feb 15:arXiv:2402.10027v1. ArXiv. 2024. Update in: Brain Stimul. 2024 May-Jun;17(3):607-615. doi: 10.1016/j.brs.2024.04.013. PMID: 38410648 Free PMC article. Updated. Preprint.
-
Device for Multifocal Delivery of Ultrasound Into Deep Brain Regions in Humans.IEEE Trans Biomed Eng. 2024 Feb;71(2):660-668. doi: 10.1109/TBME.2023.3313987. Epub 2024 Jan 19. IEEE Trans Biomed Eng. 2024. PMID: 37695955 Free PMC article.
References
-
- Boutet A, Ranjan M, Zhong J, et al. Focused ultrasound thalamotomy location determines clinical benefits in patients with essential tremor. Brain 2018;141:3405–14. - PubMed
-
- Constans C, Mateo P, Tanter M, Aubry JF. Potential impact of thermal effects during ultrasonic neurostimulation: retrospective numerical estimation of temperature elevation in seven rodent setups. Phys Med Biol 2018;63:025003. - PubMed
Publication types
MeSH terms
Grants and funding
LinkOut - more resources
Full Text Sources
Research Materials