Dietary intervention preserves β cell function in mice through CTCF-mediated transcriptional reprogramming
- PMID: 35652891
- PMCID: PMC9166293
- DOI: 10.1084/jem.20211779
Dietary intervention preserves β cell function in mice through CTCF-mediated transcriptional reprogramming
Abstract
Pancreatic β cell plasticity is the primary determinant of disease progression and remission of type 2 diabetes (T2D). However, the dynamic nature of β cell adaptation remains elusive. Here, we establish a mouse model exhibiting the compensation-to-decompensation adaptation of β cell function in response to increasing duration of high-fat diet (HFD) feeding. Comprehensive islet functional and transcriptome analyses reveal a dynamic orchestration of transcriptional networks featuring temporal alteration of chromatin remodeling. Interestingly, prediabetic dietary intervention completely rescues β cell dysfunction, accompanied by a remarkable reversal of HFD-induced reprogramming of islet chromatin accessibility and transcriptome. Mechanistically, ATAC-based motif analysis identifies CTCF as the top candidate driving dietary intervention-induced preservation of β cell function. CTCF expression is markedly decreased in β cells from obese and diabetic mice and humans. Both dietary intervention and AAV-mediated restoration of CTCF expression ameliorate β cell dysfunction ex vivo and in vivo, through transducing the lipid toxicity and inflammatory signals to transcriptional reprogramming of genes critical for β cell glucose metabolism and stress response.
© 2022 Wang et al.
Conflict of interest statement
Disclosures: The authors declare no competing interests exist.
Figures
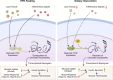
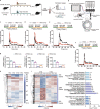
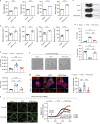
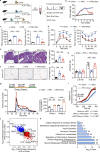
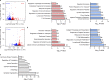
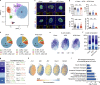
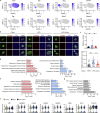
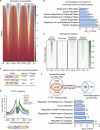
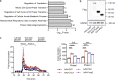
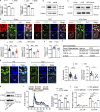
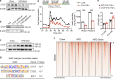
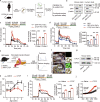
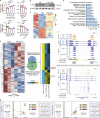
Comment in
-
Comments on 'Dietary intervention preserves β cell function in mice through CTCF-mediated transcriptional reprogramming'.J Mol Cell Biol. 2022 Sep 27;14(7):mjac043. doi: 10.1093/jmcb/mjac043. J Mol Cell Biol. 2022. PMID: 35916443 Free PMC article. No abstract available.
Similar articles
-
Metallothionein 1 negatively regulates glucose-stimulated insulin secretion and is differentially expressed in conditions of beta cell compensation and failure in mice and humans.Diabetologia. 2019 Dec;62(12):2273-2286. doi: 10.1007/s00125-019-05008-3. Epub 2019 Oct 17. Diabetologia. 2019. PMID: 31624901
-
Genome-wide profiling of histone H3K27 acetylation featured fatty acid signalling in pancreatic beta cells in diet-induced obesity in mice.Diabetologia. 2018 Dec;61(12):2608-2620. doi: 10.1007/s00125-018-4735-7. Epub 2018 Oct 3. Diabetologia. 2018. PMID: 30284014
-
Fibroblast growth factor 21 protects against lipotoxicity-induced pancreatic β-cell dysfunction via regulation of AMPK signaling and lipid metabolism.Clin Sci (Lond). 2019 Oct 15;133(19):2029-2044. doi: 10.1042/CS20190093. Clin Sci (Lond). 2019. PMID: 31654570
-
Transcriptional control of pancreatic β-cell identity and plasticity during the pathogenesis of type 2 diabetes.J Genet Genomics. 2022 Apr;49(4):316-328. doi: 10.1016/j.jgg.2022.03.002. Epub 2022 Mar 12. J Genet Genomics. 2022. PMID: 35292418 Review.
-
β-cell dynamics in type 2 diabetes and in dietary and exercise interventions.J Mol Cell Biol. 2022 Nov 30;14(7):mjac046. doi: 10.1093/jmcb/mjac046. J Mol Cell Biol. 2022. PMID: 35929791 Free PMC article. Review.
Cited by
-
Chronic Epinephrine-Induced Endoplasmic Reticulum and Oxidative Stress Impairs Pancreatic β-Cells Function and Fate.Int J Mol Sci. 2024 Jun 27;25(13):7029. doi: 10.3390/ijms25137029. Int J Mol Sci. 2024. PMID: 39000139 Free PMC article.
-
Nuclear envelope, chromatin organizers, histones, and DNA: The many achilles heels exploited across cancers.Front Cell Dev Biol. 2022 Dec 16;10:1068347. doi: 10.3389/fcell.2022.1068347. eCollection 2022. Front Cell Dev Biol. 2022. PMID: 36589746 Free PMC article. Review.
-
Metabolic regulation of CTCF expression and chromatin association dictates starvation response in mice and flies.iScience. 2023 Jun 15;26(7):107128. doi: 10.1016/j.isci.2023.107128. eCollection 2023 Jul 21. iScience. 2023. PMID: 37416476 Free PMC article.
-
The muscle-enriched myokine Musclin impairs beige fat thermogenesis and systemic energy homeostasis via Tfr1/PKA signaling in male mice.Nat Commun. 2023 Jul 19;14(1):4257. doi: 10.1038/s41467-023-39710-z. Nat Commun. 2023. PMID: 37468484 Free PMC article.
-
Epigenetic modifications in obesity-associated diseases.MedComm (2020). 2024 Feb 24;5(2):e496. doi: 10.1002/mco2.496. eCollection 2024 Feb. MedComm (2020). 2024. PMID: 38405061 Free PMC article. Review.
References
-
- Al-Mrabeh, A., Hollingsworth K.G., Shaw J.A.M., McConnachie A., Sattar N., Lean M.E.J., and Taylor R.. 2020. 2-year remission of type 2 diabetes and pancreas morphology: A post-hoc analysis of the DiRECT open-label, cluster-randomised trial. Lancet Diabetes Endocrinol. 8:939–948. 10.1016/S2213-8587(20)30303-X - DOI - PubMed
-
- Bikopoulos, G., Pimenta da Silva A., Lee S.C., Lakey J.R., Der S.D., Chan C.B., Ceddia R.B., Wheeler M.B., and Rozakis-Adcock M.. 2008. Ex vivo transcriptional profiling of human pancreatic islets following chronic exposure to monounsaturated fatty acids. J. Endocrinol. 196:455–464. 10.1677/JOE-07-0174 - DOI - PubMed
-
- Boucher, A., Lu D., Burgess S.C., Telemaque-Potts S., Jensen M.V., Mulder H., Wang M.Y., Unger R.H., Sherry A.D., and Newgard C.B.. 2004. Biochemical mechanism of lipid-induced impairment of glucose-stimulated insulin secretion and reversal with a malate analogue. J. Biol. Chem. 279:27263–27271. 10.1074/jbc.M401167200 - DOI - PubMed
-
- Brereton, M.F., Iberl M., Shimomura K., Zhang Q., Adriaenssens A.E., Proks P., Spiliotis I.I., Dace W., Mattis K.K., Ramracheya R., et al. . 2014. Reversible changes in pancreatic islet structure and function produced by elevated blood glucose. Nat. Commun. 5:4639. 10.1038/ncomms5639 - DOI - PMC - PubMed
Publication types
MeSH terms
Grants and funding
- 91857110/National Natural Science Foundation of China
- 2018YFA0800403/National Key Research and Development Programme of China
- 81722012/National Natural Science Fund for Excellent Young Scholars of China
- LZ21H070001/Zhejiang Provincial Natural Science Foundation of China
- OO20200055/Construction Fund of Key Medical Disciplines of Hangzhou
LinkOut - more resources
Full Text Sources
Other Literature Sources
Medical
Research Materials