Deciphering a hexameric protein complex with Angstrom optical resolution
- PMID: 35616526
- PMCID: PMC9142145
- DOI: 10.7554/eLife.76308
Deciphering a hexameric protein complex with Angstrom optical resolution
Abstract
Cryogenic optical localization in three dimensions (COLD) was recently shown to resolve up to four binding sites on a single protein. However, because COLD relies on intensity fluctuations that result from the blinking behavior of fluorophores, it is limited to cases where individual emitters show different brightness. This significantly lowers the measurement yield. To extend the number of resolved sites as well as the measurement yield, we employ partial labeling and combine it with polarization encoding in order to identify single fluorophores during their stochastic blinking. We then use a particle classification scheme to identify and resolve heterogenous subsets and combine them to reconstruct the three-dimensional arrangement of large molecular complexes. We showcase this method (polarCOLD) by resolving the trimer arrangement of proliferating cell nuclear antigen (PCNA) and six different sites of the hexamer protein Caseinolytic Peptidase B (ClpB) of Thermus thermophilus in its quaternary structure, both with Angstrom resolution. The combination of polarCOLD and single-particle cryogenic electron microscopy (cryoEM) promises to provide crucial insight into intrinsic heterogeneities of biomolecular structures. Furthermore, our approach is fully compatible with fluorescent protein labeling and can, thus, be used in a wide range of studies in cell and membrane biology.
Keywords: assembly; correlative imaging; cryogenic super-resolution; fluorescence; molecular biophysics; none; protein structure; structural biology.
© 2022, Mazal, Wieser et al.
Conflict of interest statement
HM, FW, VS No competing interests declared
Figures
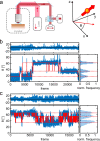
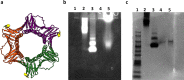
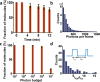
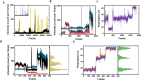
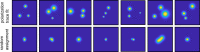
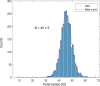
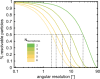
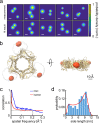
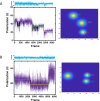
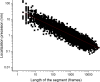
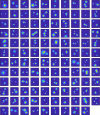
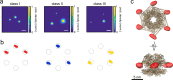
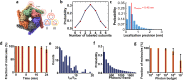
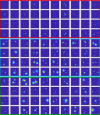
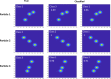
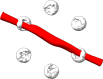
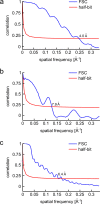
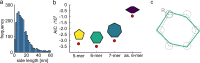
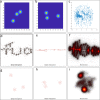
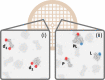
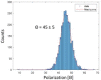
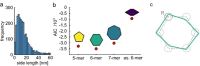
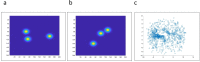
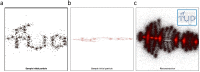
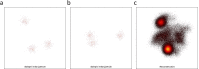
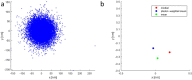
Similar articles
-
Cryogenic optical localization provides 3D protein structure data with Angstrom resolution.Nat Methods. 2017 Feb;14(2):141-144. doi: 10.1038/nmeth.4141. Epub 2017 Jan 9. Nat Methods. 2017. PMID: 28068317
-
Quantitative Single-Molecule Localization Microscopy (qSMLM) of Membrane Proteins Based on Kinetic Analysis of Fluorophore Blinking Cycles.Methods Mol Biol. 2017;1663:115-126. doi: 10.1007/978-1-4939-7265-4_10. Methods Mol Biol. 2017. PMID: 28924663
-
Long-Term, Single-Molecule Imaging of Proteins in Live Cells with Photoregulated Fluxional Fluorophores.Chemistry. 2022 Dec 20;28(71):e202202832. doi: 10.1002/chem.202202832. Epub 2022 Oct 27. Chemistry. 2022. PMID: 36125781 Free PMC article.
-
Cryogenic Super-Resolution Fluorescence and Electron Microscopy Correlated at the Nanoscale.Annu Rev Phys Chem. 2021 Apr 20;72:253-278. doi: 10.1146/annurev-physchem-090319-051546. Epub 2021 Jan 13. Annu Rev Phys Chem. 2021. PMID: 33441030 Free PMC article. Review.
-
Chemistry of Photosensitive Fluorophores for Single-Molecule Localization Microscopy.ACS Chem Biol. 2019 Jun 21;14(6):1077-1090. doi: 10.1021/acschembio.9b00197. Epub 2019 May 13. ACS Chem Biol. 2019. PMID: 30997987 Review.
Cited by
-
Characterization of mApple as a Red Fluorescent Protein for Cryogenic Single-Molecule Imaging with Turn-Off and Turn-On Active Control Mechanisms.J Phys Chem B. 2023 Mar 30;127(12):2690-2700. doi: 10.1021/acs.jpcb.2c08995. Epub 2023 Mar 21. J Phys Chem B. 2023. PMID: 36943356 Free PMC article.
-
Insights into protein structure using cryogenic light microscopy.Biochem Soc Trans. 2023 Dec 20;51(6):2041-2059. doi: 10.1042/BST20221246. Biochem Soc Trans. 2023. PMID: 38015555 Free PMC article. Review.
-
Future Paths in Cryogenic Single-Molecule Fluorescence Spectroscopy.J Phys Chem C Nanomater Interfaces. 2023 Dec 22;128(1):3-18. doi: 10.1021/acs.jpcc.3c06564. eCollection 2024 Jan 11. J Phys Chem C Nanomater Interfaces. 2023. PMID: 38229590 Free PMC article. Review.
References
-
- Agronskaia AV, Valentijn JA, van Driel LF, Schneijdenberg CTWM, Humbel BM, van Bergen en Henegouwen PMP, Verkleij AJ, Koster AJ, Gerritsen HC. Integrated fluorescence and transmission electron microscopy. Journal of Structural Biology. 2008;164:183–189. doi: 10.1016/j.jsb.2008.07.003. - DOI - PubMed
-
- Böning D, Wieser FF, Sandoghdar V. Polarization-Encoded Colocalization Microscopy at Cryogenic Temperatures. ACS Photonics. 2021;8:194–201. doi: 10.1021/acsphotonics.0c01201. - DOI
MeSH terms
Substances
Grants and funding
LinkOut - more resources
Full Text Sources
Miscellaneous