The core autophagy protein ATG9A controls dynamics of cell protrusions and directed migration
- PMID: 35180289
- PMCID: PMC8932524
- DOI: 10.1083/jcb.202106014
The core autophagy protein ATG9A controls dynamics of cell protrusions and directed migration
Abstract
Chemotactic migration is a fundamental cellular behavior relying on the coordinated flux of lipids and cargo proteins toward the leading edge. We found here that the core autophagy protein ATG9A plays a critical role in the chemotactic migration of several human cell lines, including highly invasive glioma cells. Depletion of ATG9A protein altered the formation of large and persistent filamentous actin (F-actin)-rich lamellipodia that normally drive directional migration. Using live-cell TIRF microscopy, we demonstrated that ATG9A-positive vesicles are targeted toward the migration front of polarized cells, where their exocytosis correlates with protrusive activity. Finally, we found that ATG9A was critical for efficient delivery of β1 integrin to the leading edge and normal adhesion dynamics. Collectively, our data uncover a new function for ATG9A protein and indicate that ATG9A-positive vesicles are mobilized during chemotactic stimulation to facilitate expansion of the lamellipodium and its anchorage to the extracellular matrix.
© 2022 Campisi et al.
Figures
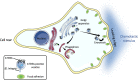
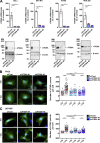
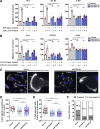
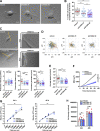
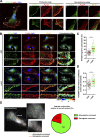
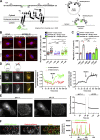
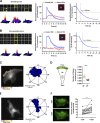
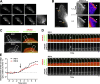
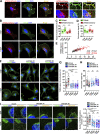
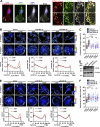
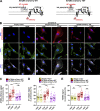
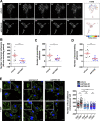
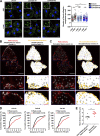
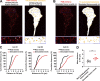
Similar articles
-
Chemotactic cell migration: the core autophagy protein ATG9A is at the leading edge.Autophagy. 2022 Dec;18(12):3037-3039. doi: 10.1080/15548627.2022.2069903. Epub 2022 Apr 29. Autophagy. 2022. PMID: 35468023 Free PMC article.
-
NMMHC IIA triggers neuronal autophagic cell death by promoting F-actin-dependent ATG9A trafficking in cerebral ischemia/reperfusion.Cell Death Dis. 2020 Jun 8;11(6):428. doi: 10.1038/s41419-020-2639-1. Cell Death Dis. 2020. PMID: 32513915 Free PMC article.
-
The autophagy protein ATG9A enables lipid mobilization from lipid droplets.Nat Commun. 2021 Nov 19;12(1):6750. doi: 10.1038/s41467-021-26999-x. Nat Commun. 2021. PMID: 34799570 Free PMC article.
-
The role of AP-4 in cargo export from the trans-Golgi network and hereditary spastic paraplegia.Biochem Soc Trans. 2020 Oct 30;48(5):1877-1888. doi: 10.1042/BST20190664. Biochem Soc Trans. 2020. PMID: 33084855 Review.
-
Steering cell migration: lamellipodium dynamics and the regulation of directional persistence.Nat Rev Mol Cell Biol. 2014 Sep;15(9):577-90. doi: 10.1038/nrm3861. Nat Rev Mol Cell Biol. 2014. PMID: 25145849 Review.
Cited by
-
ATG9A regulates the dissociation of recycling endosomes from microtubules to form liquid influenza A virus inclusions.PLoS Biol. 2023 Nov 20;21(11):e3002290. doi: 10.1371/journal.pbio.3002290. eCollection 2023 Nov. PLoS Biol. 2023. PMID: 37983294 Free PMC article.
-
Emerging roles of ATG9/ATG9A in autophagy: implications for cell and neurobiology.Autophagy. 2024 Nov;20(11):2373-2387. doi: 10.1080/15548627.2024.2384349. Epub 2024 Aug 4. Autophagy. 2024. PMID: 39099167 Free PMC article. Review.
-
Integrating intracellular nanovesicles into integrin trafficking pathways and beyond.Cell Mol Life Sci. 2022 Jun 3;79(6):335. doi: 10.1007/s00018-022-04371-6. Cell Mol Life Sci. 2022. PMID: 35657500 Free PMC article. Review.
-
Biogenesis of omegasomes and autophagosomes in mammalian autophagy.Biochem Soc Trans. 2024 Oct 30;52(5):2145-2155. doi: 10.1042/BST20240015. Biochem Soc Trans. 2024. PMID: 39392358 Free PMC article. Review.
-
Autophagy-associated immune dysregulation and hyperplasia in a patient with compound heterozygous mutations in ATG9A.Autophagy. 2023 Feb;19(2):678-691. doi: 10.1080/15548627.2022.2093028. Epub 2022 Jul 15. Autophagy. 2023. PMID: 35838483 Free PMC article.
References
-
- Adelmann-Grill, B.C., Wach F., Cully Z., Hein R., and Krieg T.. 1990. Chemotactic migration of normal dermal fibroblasts towards epidermal growth factor and its modulation by platelet-derived growth factor and transforming growth factor-beta. Eur. J. Cell Biol. 51:322–326. - PubMed
Publication types
MeSH terms
Substances
LinkOut - more resources
Full Text Sources
Molecular Biology Databases
Research Materials