Conformational Dynamics of Intrinsically Disordered Proteins Regulate Biomolecular Condensate Chemistry
- PMID: 35179885
- PMCID: PMC8949871
- DOI: 10.1021/acs.chemrev.1c00774
Conformational Dynamics of Intrinsically Disordered Proteins Regulate Biomolecular Condensate Chemistry
Abstract
Motions in biomolecules are critical for biochemical reactions. In cells, many biochemical reactions are executed inside of biomolecular condensates formed by ultradynamic intrinsically disordered proteins. A deep understanding of the conformational dynamics of intrinsically disordered proteins in biomolecular condensates is therefore of utmost importance but is complicated by diverse obstacles. Here we review emerging data on the motions of intrinsically disordered proteins inside of liquidlike condensates. We discuss how liquid-liquid phase separation modulates internal motions across a wide range of time and length scales. We further highlight the importance of intermolecular interactions that not only drive liquid-liquid phase separation but appear as key determinants for changes in biomolecular motions and the aging of condensates in human diseases. The review provides a framework for future studies to reveal the conformational dynamics of intrinsically disordered proteins in the regulation of biomolecular condensate chemistry.
Conflict of interest statement
The authors declare no competing financial interest.
Figures
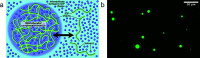
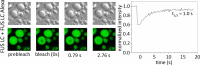
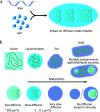
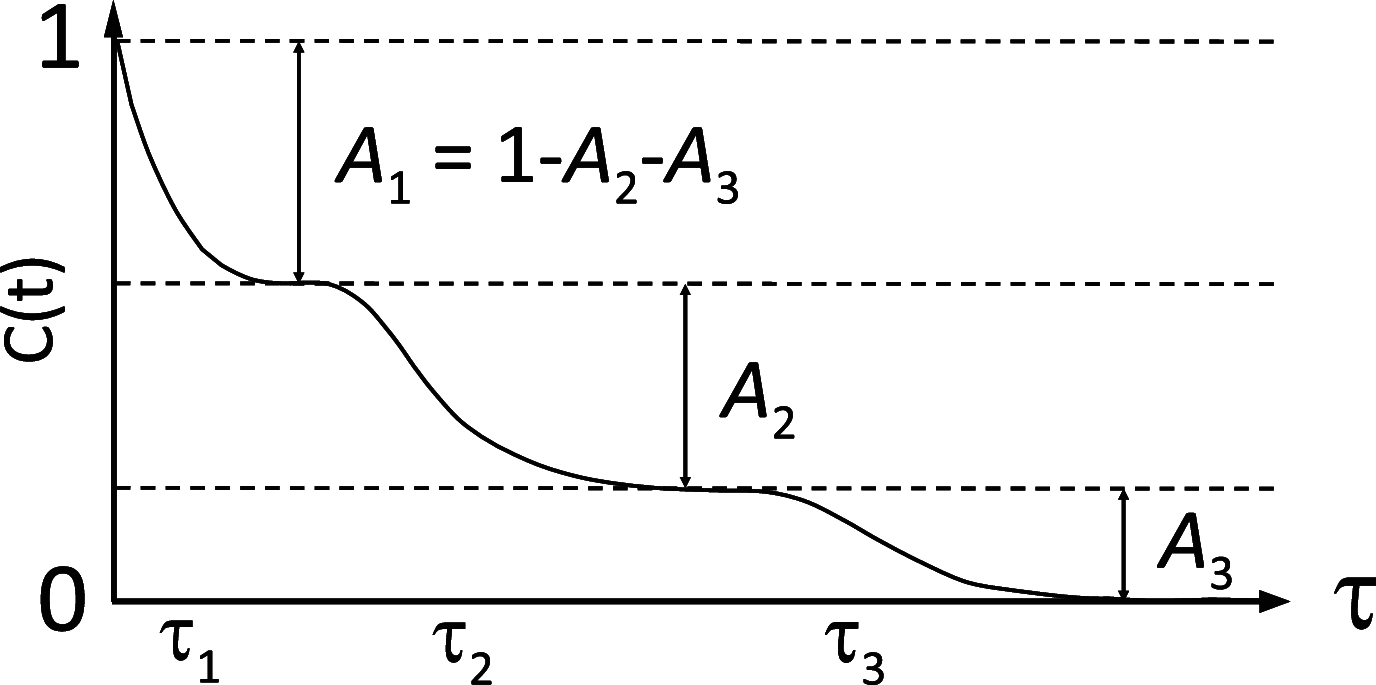
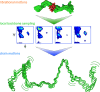
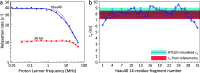
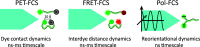
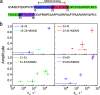
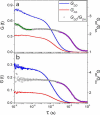
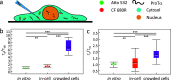
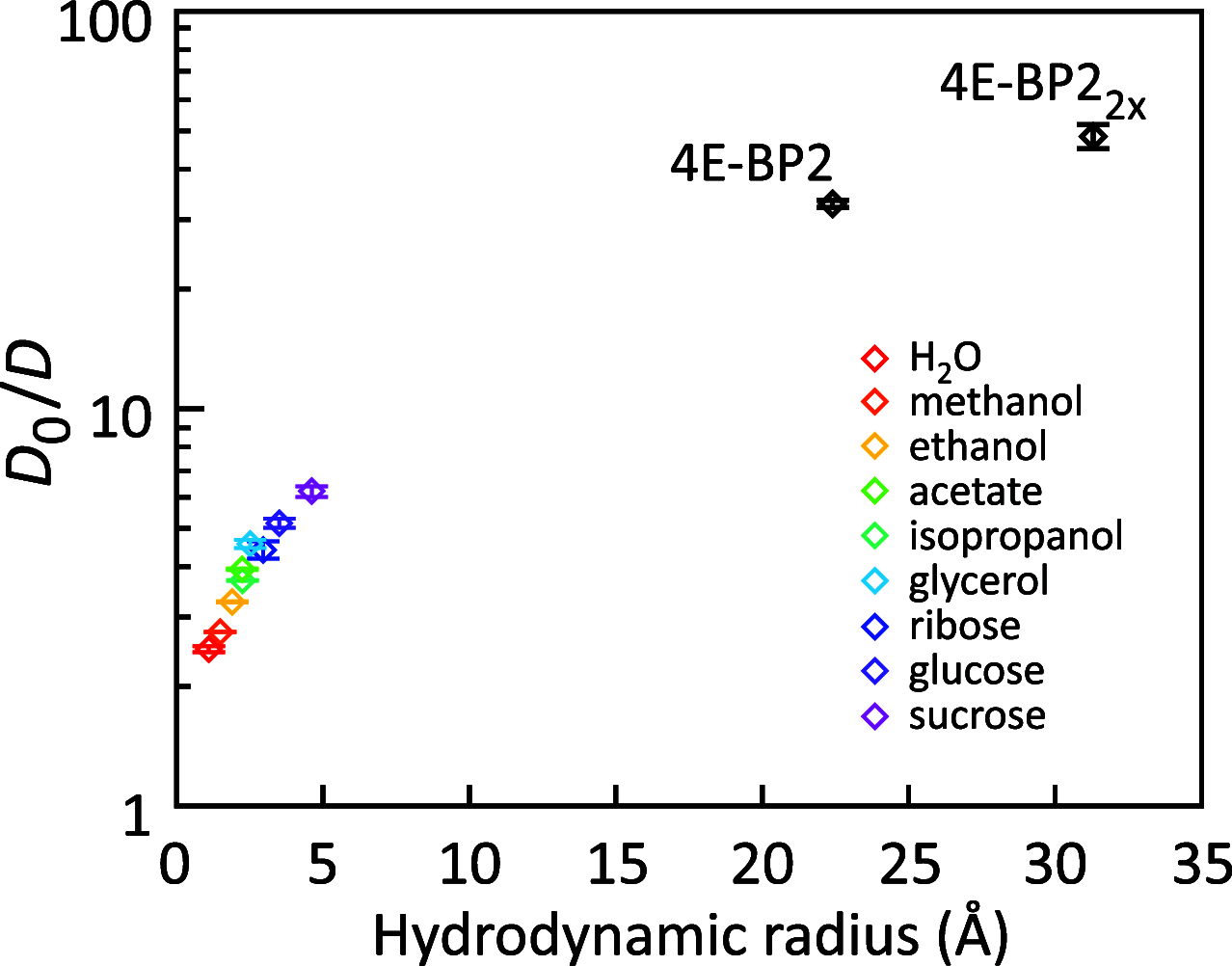
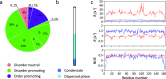
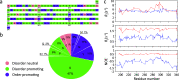
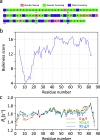
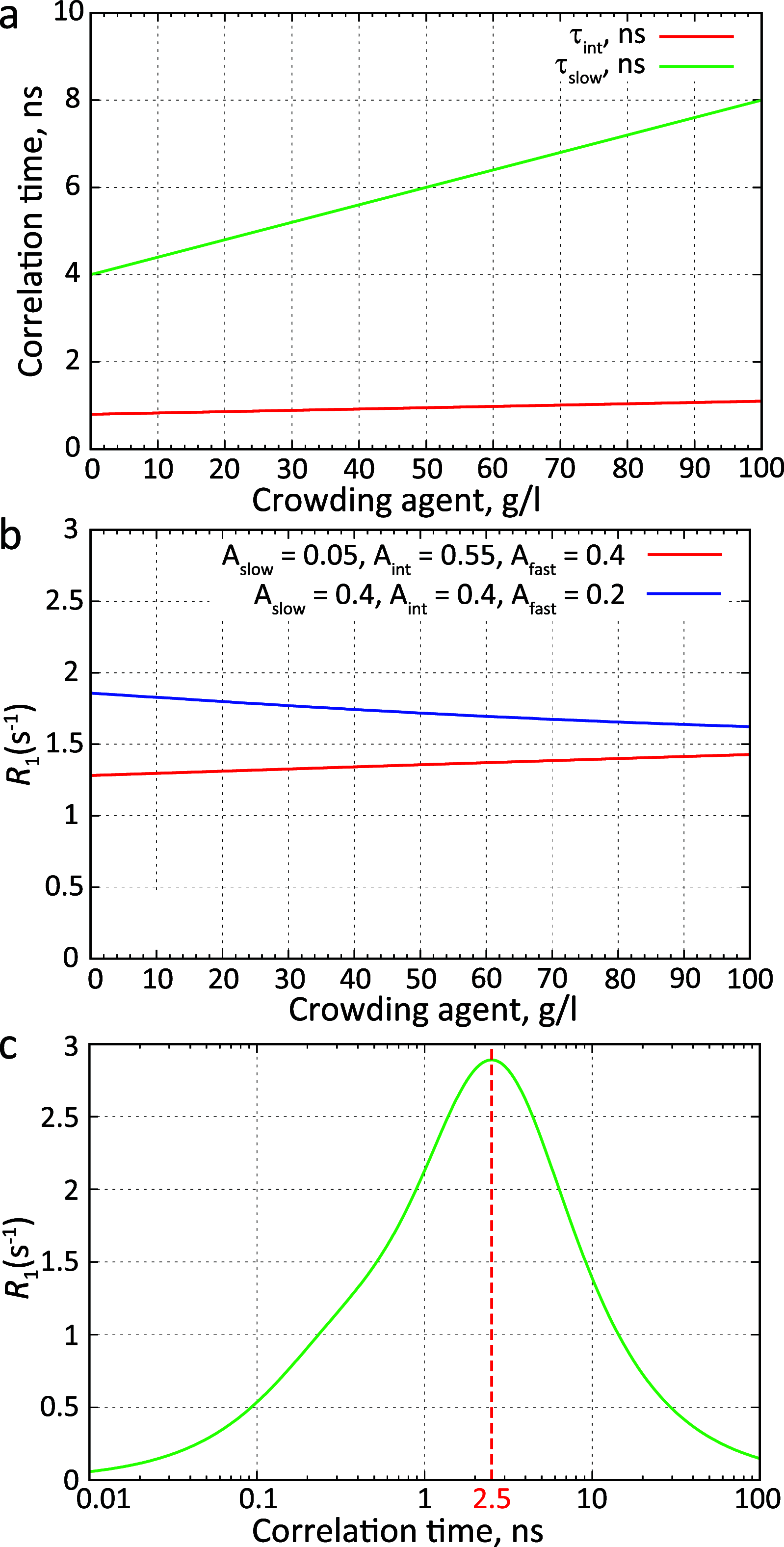
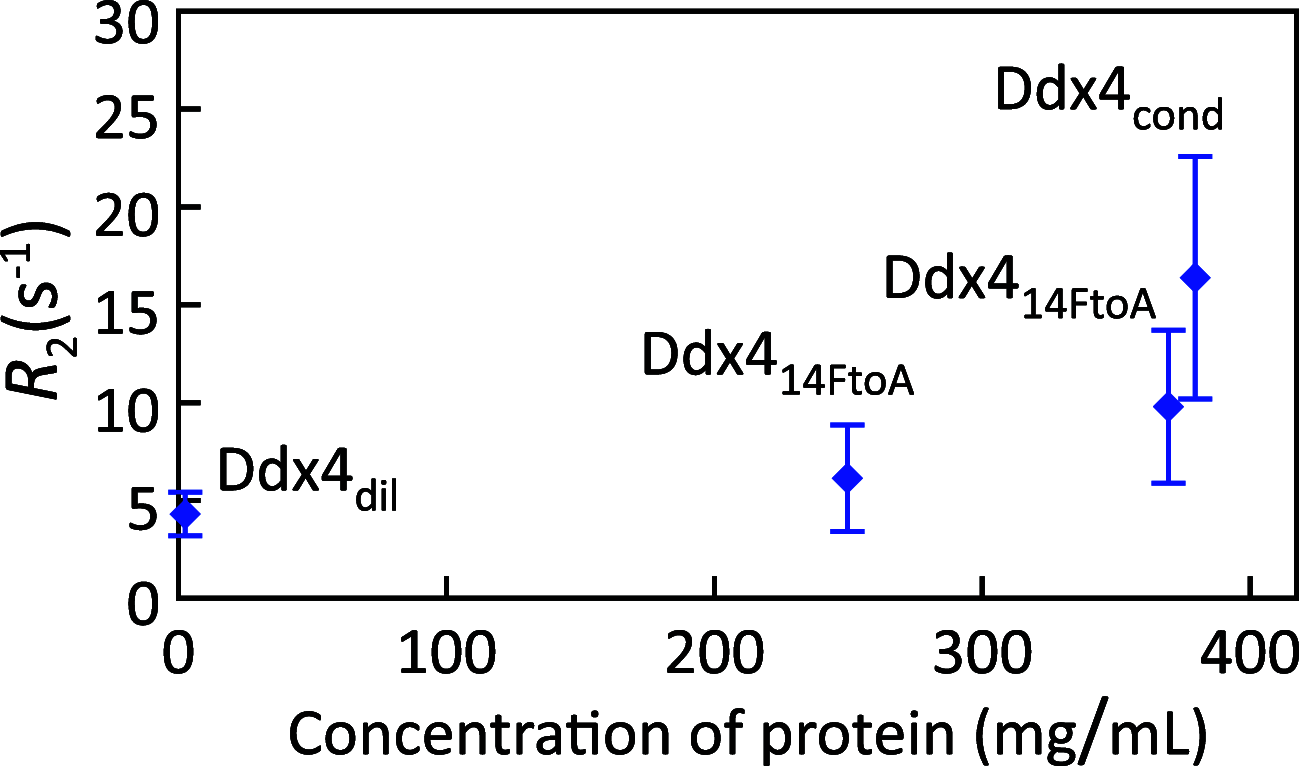
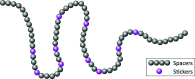
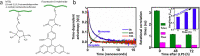
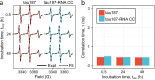
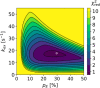
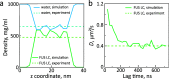
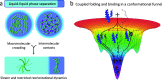
Similar articles
-
Heterogeneous Slowdown of Dynamics in the Condensate of an Intrinsically Disordered Protein.J Phys Chem Lett. 2024 Nov 14;15(45):11244-11251. doi: 10.1021/acs.jpclett.4c02142. Epub 2024 Nov 1. J Phys Chem Lett. 2024. PMID: 39486437 Free PMC article.
-
A call to order: Examining structured domains in biomolecular condensates.J Magn Reson. 2023 Jan;346:107318. doi: 10.1016/j.jmr.2022.107318. J Magn Reson. 2023. PMID: 36657879 Free PMC article.
-
Unravelling the microscopic characteristics of intrinsically disordered proteins upon liquid-liquid phase separation.Essays Biochem. 2022 Dec 16;66(7):891-900. doi: 10.1042/EBC20220148. Essays Biochem. 2022. PMID: 36524527 Review.
-
Sequence determinants of in cell condensate morphology, dynamics, and oligomerization as measured by number and brightness analysis.Cell Commun Signal. 2021 Jun 5;19(1):65. doi: 10.1186/s12964-021-00744-9. Cell Commun Signal. 2021. PMID: 34090478 Free PMC article.
-
Biomolecular condensates in cancer biology.Cancer Sci. 2022 Feb;113(2):382-391. doi: 10.1111/cas.15232. Epub 2021 Dec 14. Cancer Sci. 2022. PMID: 34865286 Free PMC article. Review.
Cited by
-
BEMM-GEN: A Toolkit for Generating a Biomolecular Environment-Mimicking Model for Molecular Dynamics Simulation.J Chem Inf Model. 2024 Oct 14;64(19):7184-7188. doi: 10.1021/acs.jcim.4c01467. Epub 2024 Oct 3. J Chem Inf Model. 2024. PMID: 39361452 Free PMC article.
-
Progress, Challenges and Opportunities of NMR and XL-MS for Cellular Structural Biology.JACS Au. 2024 Feb 5;4(2):369-383. doi: 10.1021/jacsau.3c00712. eCollection 2024 Feb 26. JACS Au. 2024. PMID: 38425916 Free PMC article. Review.
-
From the Catastrophic Objective Irreproducibility of Cancer Research and Unavoidable Failures of Molecular Targeted Therapies to the Sparkling Hope of Supramolecular Targeted Strategies.Int J Mol Sci. 2023 Feb 1;24(3):2796. doi: 10.3390/ijms24032796. Int J Mol Sci. 2023. PMID: 36769134 Free PMC article. Review.
-
Stress-related biomolecular condensates in plants.Plant Cell. 2023 Sep 1;35(9):3187-3204. doi: 10.1093/plcell/koad127. Plant Cell. 2023. PMID: 37162152 Free PMC article.
-
Diversity of hydrodynamic radii of intrinsically disordered proteins.Eur Biophys J. 2023 Oct;52(6-7):607-618. doi: 10.1007/s00249-023-01683-8. Epub 2023 Oct 13. Eur Biophys J. 2023. PMID: 37831084 Free PMC article.
References
-
- Alberts B.Molecular Biology of the Cell; Garland Science, 2017; pp 8–9.
Publication types
MeSH terms
Substances
LinkOut - more resources
Full Text Sources