N-terminal phosphorylation regulates the activity of glycogen synthase kinase 3 from Plasmodium falciparum
- PMID: 35023554
- PMCID: PMC8883495
- DOI: 10.1042/BCJ20210829
N-terminal phosphorylation regulates the activity of glycogen synthase kinase 3 from Plasmodium falciparum
Abstract
As the decline of malaria cases stalled over the last five years, novel targets in Plasmodium falciparum are necessary for the development of new drugs. Glycogen Synthase Kinase (PfGSK3) has been identified as a potential target, since its selective inhibitors were shown to disrupt the parasitès life cycle. In the uncanonical N-terminal region of the parasite enzyme, we identified several autophosphorylation sites and probed their role in activity regulation of PfGSK3. By combining molecular modeling with experimental small-angle X-ray scattering data, we show that increased PfGSK3 activity is promoted by conformational changes in the PfGSK3 N-terminus, triggered by N-terminal phosphorylation. Our work provides novel insights into the structure and regulation of the malarial PfGSK3.
Keywords: autophosphorylation; drug target; glycogen synthase kinase; malaria; small-angle scattering.
© 2022 The Author(s).
Conflict of interest statement
The authors declare that there are no competing interests associated with the manuscript.
Figures
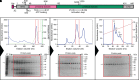
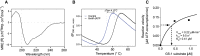
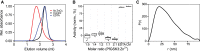
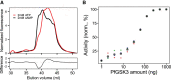
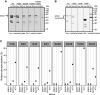
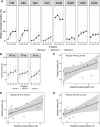
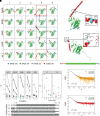
Similar articles
-
Functional inactivation of Plasmodium falciparum glycogen synthase kinase GSK3 modulates erythrocyte invasion and blocks gametocyte maturation.J Biol Chem. 2022 Sep;298(9):102360. doi: 10.1016/j.jbc.2022.102360. Epub 2022 Aug 10. J Biol Chem. 2022. PMID: 35961464 Free PMC article.
-
Identification of Novel 2,4,5-Trisubstituted Pyrimidines as Potent Dual Inhibitors of Plasmodial PfGSK3/PfPK6 with Activity against Blood Stage Parasites In Vitro.J Med Chem. 2022 Oct 13;65(19):13172-13197. doi: 10.1021/acs.jmedchem.2c00996. Epub 2022 Sep 27. J Med Chem. 2022. PMID: 36166733 Free PMC article.
-
Global kinomic and phospho-proteomic analyses of the human malaria parasite Plasmodium falciparum.Nat Commun. 2011 Nov 29;2:565. doi: 10.1038/ncomms1558. Nat Commun. 2011. PMID: 22127061
-
Selective inhibitors of Plasmodium falciparum glycogen synthase-3 (PfGSK-3): New antimalarial agents?Biochim Biophys Acta. 2015 Oct;1854(10 Pt B):1644-9. doi: 10.1016/j.bbapap.2015.03.013. Epub 2015 Apr 7. Biochim Biophys Acta. 2015. PMID: 25861860 Review.
-
Plasmodium falciparum glycogen synthase kinase-3: molecular model, expression, intracellular localisation and selective inhibitors.Biochim Biophys Acta. 2004 Mar 11;1697(1-2):181-96. doi: 10.1016/j.bbapap.2003.11.023. Biochim Biophys Acta. 2004. PMID: 15023360 Review.
References
-
- World Health Organization. (2019). World Malaria Report. https://www.who.int/malaria. (accessed 15 Dec. 2019). Available from: https://www.who.int/publications-detail/world-malaria-report-2019
Publication types
MeSH terms
Substances
LinkOut - more resources
Full Text Sources
Research Materials