Structure, Function, and Pharmacology of Glutamate Receptor Ion Channels
- PMID: 34753794
- PMCID: PMC8626789
- DOI: 10.1124/pharmrev.120.000131
Structure, Function, and Pharmacology of Glutamate Receptor Ion Channels
Abstract
Many physiologic effects of l-glutamate, the major excitatory neurotransmitter in the mammalian central nervous system, are mediated via signaling by ionotropic glutamate receptors (iGluRs). These ligand-gated ion channels are critical to brain function and are centrally implicated in numerous psychiatric and neurologic disorders. There are different classes of iGluRs with a variety of receptor subtypes in each class that play distinct roles in neuronal functions. The diversity in iGluR subtypes, with their unique functional properties and physiologic roles, has motivated a large number of studies. Our understanding of receptor subtypes has advanced considerably since the first iGluR subunit gene was cloned in 1989, and the research focus has expanded to encompass facets of biology that have been recently discovered and to exploit experimental paradigms made possible by technological advances. Here, we review insights from more than 3 decades of iGluR studies with an emphasis on the progress that has occurred in the past decade. We cover structure, function, pharmacology, roles in neurophysiology, and therapeutic implications for all classes of receptors assembled from the subunits encoded by the 18 ionotropic glutamate receptor genes. SIGNIFICANCE STATEMENT: Glutamate receptors play important roles in virtually all aspects of brain function and are either involved in mediating some clinical features of neurological disease or represent a therapeutic target for treatment. Therefore, understanding the structure, function, and pharmacology of this class of receptors will advance our understanding of many aspects of brain function at molecular, cellular, and system levels and provide new opportunities to treat patients.
U.S. Government work not protected by U.S. copyright.
Figures
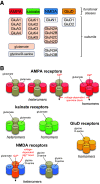
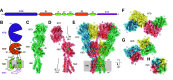
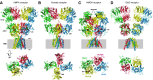
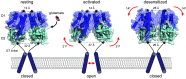
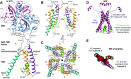
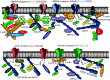
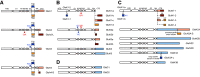
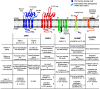
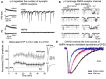
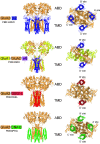
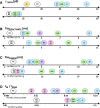
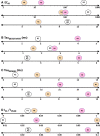
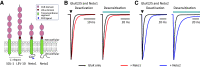
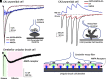
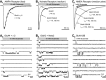
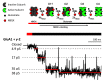
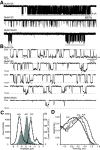
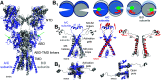
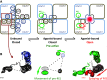
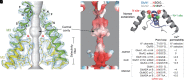
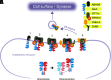
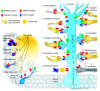
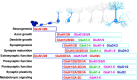
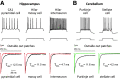
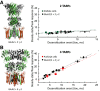
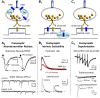
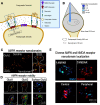
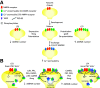
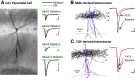
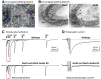
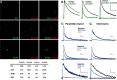
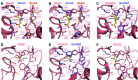
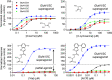
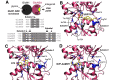
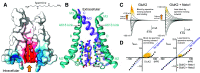
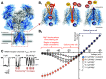
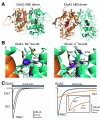
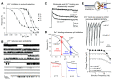
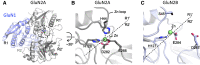
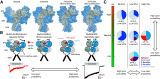
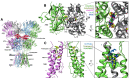
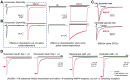
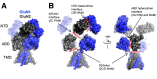
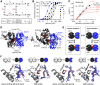
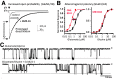
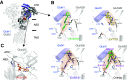
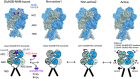
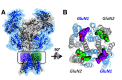
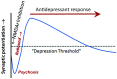
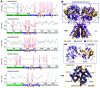
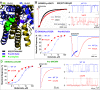
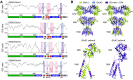
Similar articles
-
A Molecular Determinant of Subtype-Specific Desensitization in Ionotropic Glutamate Receptors.J Neurosci. 2016 Mar 2;36(9):2617-22. doi: 10.1523/JNEUROSCI.2667-15.2016. J Neurosci. 2016. PMID: 26937003 Free PMC article.
-
Glycine agonism in ionotropic glutamate receptors.Neuropharmacology. 2021 Aug 1;193:108631. doi: 10.1016/j.neuropharm.2021.108631. Epub 2021 May 28. Neuropharmacology. 2021. PMID: 34058193 Review.
-
Glutamatergic Signaling in the Central Nervous System: Ionotropic and Metabotropic Receptors in Concert.Neuron. 2018 Jun 27;98(6):1080-1098. doi: 10.1016/j.neuron.2018.05.018. Neuron. 2018. PMID: 29953871 Free PMC article. Review.
-
[Excitatory amino acid receptors].Nihon Yakurigaku Zasshi. 1994 Sep;104(3):177-87. doi: 10.1254/fpj.104.177. Nihon Yakurigaku Zasshi. 1994. PMID: 7959410 Review. Japanese.
-
New insights into the structure and function of glutamate receptors: the orphan receptor delta2 reveals its family's secrets.Keio J Med. 2003 Jun;52(2):92-9. doi: 10.2302/kjm.52.92. Keio J Med. 2003. PMID: 12862360 Review.
Cited by
-
Mechanistic and structural studies reveal NRAP-1-dependent coincident activation of NMDARs.Cell Rep. 2024 Feb 27;43(2):113694. doi: 10.1016/j.celrep.2024.113694. Epub 2024 Jan 23. Cell Rep. 2024. PMID: 38265937 Free PMC article.
-
Behavioral analysis of kainate receptor KO mice and the role of GluK3 subunit in anxiety.Sci Rep. 2024 Feb 24;14(1):4521. doi: 10.1038/s41598-024-55063-z. Sci Rep. 2024. PMID: 38402313 Free PMC article.
-
Hsp47 promotes biogenesis of multi-subunit neuroreceptors in the endoplasmic reticulum.Elife. 2024 Jul 4;13:e84798. doi: 10.7554/eLife.84798. Elife. 2024. PMID: 38963323 Free PMC article.
-
Effects of ethanol on GluN1/GluN2A and GluN1/GluN2B NMDA receptor-ion channel gating kinetics.Alcohol Clin Exp Res. 2022 Dec;46(12):2203-2213. doi: 10.1111/acer.14965. Epub 2022 Nov 9. Alcohol Clin Exp Res. 2022. PMID: 36305341 Free PMC article.
-
Synapse Dysfunctions in Multiple Sclerosis.Int J Mol Sci. 2023 Jan 13;24(2):1639. doi: 10.3390/ijms24021639. Int J Mol Sci. 2023. PMID: 36675155 Free PMC article. Review.
References
-
- Aamodt SM, Shi J, Colonnese MT, Veras W, Constantine-Paton M (2000) Chronic NMDA exposure accelerates development of GABAergic inhibition in the superior colliculus. J Neurophysiol 83:1580–1591. - PubMed
-
- aan het Rot M, Collins KA, Murrough JW, Perez AM, Reich DL, Charney DS, Mathew SJ (2010) Safety and efficacy of repeated-dose intravenous ketamine for treatment-resistant depression. Biol Psychiatry 67:139–145. - PubMed
-
- Abbott LF, Nelson SB (2000) Synaptic plasticity: taming the beast. Nat Neurosci 3 (Suppl):1178–1183. - PubMed
Publication types
MeSH terms
Substances
Grants and funding
- R01 MH085926/MH/NIMH NIH HHS/United States
- R01 NS107253/NS/NINDS NIH HHS/United States
- R01 NS088479/NS/NINDS NIH HHS/United States
- MC_U105174197/MRC_/Medical Research Council/United Kingdom
- R01 NS040701/NS/NINDS NIH HHS/United States
- RF1 NS113632/NS/NINDS NIH HHS/United States
- R01 NS105804/NS/NINDS NIH HHS/United States
- R35 GM122528/GM/NIGMS NIH HHS/United States
- R01 NS116055/NS/NINDS NIH HHS/United States
- R01 NS097536/NS/NINDS NIH HHS/United States
- R01 MH123474/MH/NIMH NIH HHS/United States
- R37 NS083660/NS/NINDS NIH HHS/United States
- R56 MH123474/MH/NIMH NIH HHS/United States
- R01 NS105502/NS/NINDS NIH HHS/United States
- R01 NS111745/NS/NINDS NIH HHS/United States
- R56 NS040701/NS/NINDS NIH HHS/United States
- R01 HD082373/HD/NICHD NIH HHS/United States
- F31 NS113530/NS/NINDS NIH HHS/United States
- BB/N002113/1/BB_/Biotechnology and Biological Sciences Research Council/United Kingdom
- P20 GM103546/GM/NIGMS NIH HHS/United States
- R01 NS083660/NS/NINDS NIH HHS/United States
- R35 NS111619/NS/NINDS NIH HHS/United States
- R21 MH127404/MH/NIMH NIH HHS/United States
- R01 CA206573/CA/NCI NIH HHS/United States
LinkOut - more resources
Full Text Sources
Other Literature Sources
Miscellaneous