Electrode Materials for Chronic Electrical Microstimulation
- PMID: 34029008
- PMCID: PMC8257249
- DOI: 10.1002/adhm.202100119
Electrode Materials for Chronic Electrical Microstimulation
Abstract
Electrical microstimulation has enabled partial restoration of vision, hearing, movement, somatosensation, as well as improving organ functions by electrically modulating neural activities. However, chronic microstimulation is faced with numerous challenges. The implantation of an electrode array into the neural tissue triggers an inflammatory response, which can be exacerbated by the delivery of electrical currents. Meanwhile, prolonged stimulation may lead to electrode material degradation., which can be accelerated by the hostile inflammatory environment. Both material degradation and adverse tissue reactions can compromise stimulation performance over time. For stable chronic electrical stimulation, an ideal microelectrode must present 1) high charge injection limit, to efficiently deliver charge without exceeding safety limits for both tissue and electrodes, 2) small size, to gain high spatial selectivity, 3) excellent biocompatibility that ensures tissue health immediately next to the device, and 4) stable in vivo electrochemical properties over the application period. In this review, the challenges in chronic microstimulation are described in detail. To aid material scientists interested in neural stimulation research, the in vitro and in vivo testing methods are introduced for assessing stimulation functionality and longevity and a detailed overview of recent advances in electrode material research and device fabrication for improving chronic microstimulation performance is provided.
Keywords: carbon materials; chronic neural stimulation; conducting polymers; metals.
© 2021 Wiley-VCH GmbH.
Figures
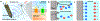
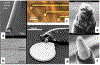
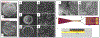
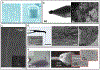
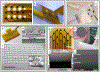
Similar articles
-
Ultrasoft microwire neural electrodes improve chronic tissue integration.Acta Biomater. 2017 Apr 15;53:46-58. doi: 10.1016/j.actbio.2017.02.010. Epub 2017 Feb 6. Acta Biomater. 2017. PMID: 28185910 Free PMC article.
-
Polydopamine-doped conductive polymer microelectrodes for neural recording and stimulation.J Neurosci Methods. 2019 Oct 1;326:108369. doi: 10.1016/j.jneumeth.2019.108369. Epub 2019 Jul 18. J Neurosci Methods. 2019. PMID: 31326604
-
Effects of Synchronous Electrode Pulses on Neural Recruitment During Multichannel Microstimulation.Sci Rep. 2018 Aug 30;8(1):13067. doi: 10.1038/s41598-018-31247-2. Sci Rep. 2018. PMID: 30166583 Free PMC article.
-
Neural stimulation and recording electrodes.Annu Rev Biomed Eng. 2008;10:275-309. doi: 10.1146/annurev.bioeng.10.061807.160518. Annu Rev Biomed Eng. 2008. PMID: 18429704 Review.
-
Organic electrode coatings for next-generation neural interfaces.Front Neuroeng. 2014 May 27;7:15. doi: 10.3389/fneng.2014.00015. eCollection 2014. Front Neuroeng. 2014. PMID: 24904405 Free PMC article. Review.
Cited by
-
Nanocone-Array-Based Platinum-Iridium Oxide Neural Microelectrodes: Structure, Electrochemistry, Durability and Biocompatibility Study.Nanomaterials (Basel). 2022 Oct 1;12(19):3445. doi: 10.3390/nano12193445. Nanomaterials (Basel). 2022. PMID: 36234573 Free PMC article.
-
Nanomaterial-based microelectrode arrays for in vitro bidirectional brain-computer interfaces: a review.Microsyst Nanoeng. 2023 Jan 30;9:13. doi: 10.1038/s41378-022-00479-8. eCollection 2023. Microsyst Nanoeng. 2023. PMID: 36726940 Free PMC article. Review.
-
PEDOT:PSS-coated platinum electrodes for neural stimulation.APL Bioeng. 2023 Dec 5;7(4):046117. doi: 10.1063/5.0153094. eCollection 2023 Dec. APL Bioeng. 2023. PMID: 38075207 Free PMC article.
-
Computational analysis of electrode structure and configuration for efficient and localized neural stimulation.Biomed Eng Lett. 2024 Mar 16;14(4):717-726. doi: 10.1007/s13534-024-00364-5. eCollection 2024 Jul. Biomed Eng Lett. 2024. PMID: 38946826
-
Effects of central nervous system electrical stimulation on non-neuronal cells.Front Neurosci. 2022 Sep 15;16:967491. doi: 10.3389/fnins.2022.967491. eCollection 2022. Front Neurosci. 2022. PMID: 36188481 Free PMC article. Review.
References
-
- Yeomans JS, Frankland PW, Brain research reviews 1995, 21, 301. - PubMed
-
- Tehovnik EJ, Slocum WM, Schiller PH, European Journal of Neuroscience 2004, 20, 264. - PubMed
-
- Strick PL, Preston JB, Brain research 1978, 154, 366. - PubMed
-
- Schmidt E, Bak M, Hambrecht F, Kufta C, O’rourke D, Vallabhanath P, Brain 1996, 119, 507. - PubMed
-
- McCreery D, Yuen T, Bullara L, Hearing Research 2000, 149, 223. - PubMed
Publication types
MeSH terms
Grants and funding
LinkOut - more resources
Full Text Sources
Other Literature Sources