3D Encapsulation and tethering of functionally engineered extracellular vesicles to hydrogels
- PMID: 33741538
- PMCID: PMC8096714
- DOI: 10.1016/j.actbio.2021.03.030
3D Encapsulation and tethering of functionally engineered extracellular vesicles to hydrogels
Abstract
Mesenchymal stem cell (MSC) derived extracellular vesicles (EVs) in their naïve and engineered forms have emerged as potential alternatives to stem cell therapy. While they have a defined therapeutic potential, the spatial and temporal control of their activity in vivo remains a challenge. The objective of this study was to devise a methodology to encapsulate EVs in 3D hydrogels for prolonged delivery. To achieve this, we have leveraged the MSC EV interactions with ECM proteins and their derivative peptides. Using osteoinductive functionally engineered EVs (FEEs) derived from MSCs, we show that FEEs bind to mimetic peptides from collagen (DGEA, GFPGER) and fibronectin (RGD). In in vitro experiments, photocrosslinkable alginate hydrogels containing RGD were able to encapsulate, tether and retain the FEEs over a period of 7 days while maintaining the structural integrity and osteoinductive functionality of the EVs. When employed in a calvarial defect model in vivo, alginate-RGD hydrogels containing the FEEs enhanced bone regeneration by a factor of 4 compared to controls lacking FEEs and by a factor of 2 compared to controls lacking the tethering peptide. These results show that EVs can be tethered to biomaterials to promote bone repair and the importance of prolonged delivery in vivo. Results also provide a prelude to the possible use of this technology for controlled delivery of EVs for other regenerative medicine applications. STATEMENT OF SIGNIFICANCE: The beneficial effects of human MSC (HMSC) therapy are attributable to paracrine effects of the HMSC derived EVs. While EV engineering has the potential to impact several fields of regenerative medicine, targeted delivery of the engineered EVs with spatial and temporal control is necessary to prevent off-target effects and enhance tissue specificity. Here, we have leveraged the interactions of MSC EVs with ECM proteins to develop a tethering system that can be utilized to prolong EV delivery in vivo while maintaining the structural and functional integrity of the EVs. Our work has provided a tunable platform for EV delivery that we envision can be formulated as an injectable material or a bulk hydrogel suitable for regenerative medicine applications.
Keywords: Alginate; Bone regeneration; Controlled release; Exosomes; Extracellular vesicles; Integrins.
Copyright © 2021. Published by Elsevier Ltd.
Conflict of interest statement
Declaration of Competing Interest The authors declare that they have no known competing financial interests or personal relationships that could have appeared to influence the work reported in this paper.
Figures
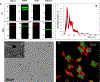
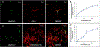
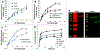
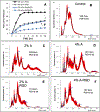
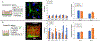
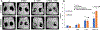
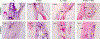
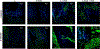
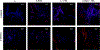
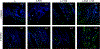
Similar articles
-
Functionally engineered extracellular vesicles improve bone regeneration.Acta Biomater. 2020 Jun;109:182-194. doi: 10.1016/j.actbio.2020.04.017. Epub 2020 Apr 16. Acta Biomater. 2020. PMID: 32305445 Free PMC article.
-
Extracellular vesicle-loaded hydrogels for tissue repair and regeneration.Mater Today Bio. 2022 Dec 21;18:100522. doi: 10.1016/j.mtbio.2022.100522. eCollection 2023 Feb. Mater Today Bio. 2022. PMID: 36593913 Free PMC article. Review.
-
Hydrogel-Assisted 3D Model to Investigate the Osteoinductive Potential of MC3T3-Derived Extracellular Vesicles.ACS Biomater Sci Eng. 2021 Jun 14;7(6):2687-2700. doi: 10.1021/acsbiomaterials.1c00386. Epub 2021 May 21. ACS Biomater Sci Eng. 2021. PMID: 34018721
-
Controlled Release of Epigenetically-Enhanced Extracellular Vesicles from a GelMA/Nanoclay Composite Hydrogel to Promote Bone Repair.Int J Mol Sci. 2022 Jan 13;23(2):832. doi: 10.3390/ijms23020832. Int J Mol Sci. 2022. PMID: 35055017 Free PMC article.
-
Mesenchymal Stem Cell Derived Extracellular Vesicles for Tissue Engineering and Regenerative Medicine Applications.Cells. 2020 Apr 16;9(4):991. doi: 10.3390/cells9040991. Cells. 2020. PMID: 32316248 Free PMC article. Review.
Cited by
-
Extracellular Vesicles and Hydrogels: An Innovative Approach to Tissue Regeneration.ACS Omega. 2024 Jan 31;9(6):6184-6218. doi: 10.1021/acsomega.3c08280. eCollection 2024 Feb 13. ACS Omega. 2024. PMID: 38371801 Free PMC article. Review.
-
Micro RNA based MSC EV engineering: Targeting the BMP2 cascade for bone repair.Front Cell Dev Biol. 2023 Feb 8;11:1127594. doi: 10.3389/fcell.2023.1127594. eCollection 2023. Front Cell Dev Biol. 2023. PMID: 36846585 Free PMC article.
-
Tissue Engineering Supporting Regenerative Strategies to Enhance Clinical Orthodontics and Dentofacial Orthopaedics: A Scoping, Perspective Review.Biomedicines. 2023 Mar 6;11(3):795. doi: 10.3390/biomedicines11030795. Biomedicines. 2023. PMID: 36979774 Free PMC article. Review.
-
Extracellular vesicles: From bone development to regenerative orthopedics.Mol Ther. 2023 May 3;31(5):1251-1274. doi: 10.1016/j.ymthe.2023.02.021. Epub 2023 Mar 3. Mol Ther. 2023. PMID: 36869588 Free PMC article. Review.
-
Digital Light Processing 3D Bioprinting of Gelatin-Norbornene Hydrogel for Enhanced Vascularization.Macromol Biosci. 2023 Dec;23(12):e2300213. doi: 10.1002/mabi.202300213. Epub 2023 Aug 9. Macromol Biosci. 2023. PMID: 37536347 Free PMC article.
References
-
- Park IH, Micic ID, Jeon IH, A study of 23 unicameral bone cysts of the calcaneus: open chip allogeneic bone graft versus percutaneous injection of bone powder with autogenous bone marrow, Foot & ankle international 29(2) (2008) 164–70. - PubMed
-
- Johnstone RM, Mathew A, Mason AB, Teng K, Exosome formation during maturation of mammalian and avian reticulocytes: evidence that exosome release is a major route for externalization of obsolete membrane proteins, Journal of cellular physiology 147(1) (1991) 27–36. - PubMed
-
- Svensson KJ, Christianson HC, Wittrup A, Bourseau-Guilmain E, Lindqvist E, Svensson LM, Morgelin M, Belting M, Exosome uptake depends on ERK1/2-heat shock protein 27 signaling and lipid Raft-mediated endocytosis negatively regulated by caveolin-1, The Journal of biological chemistry 288(24) (2013) 17713–24. - PMC - PubMed
-
- Valadi H, Ekstrom K, Bossios A, Sjostrand M, Lee JJ, Lotvall JO, Exosome-mediated transfer of mRNAs and microRNAs is a novel mechanism of genetic exchange between cells, Nat Cell Biol 9(6) (2007) 654–9. - PubMed
Publication types
MeSH terms
Substances
Grants and funding
LinkOut - more resources
Full Text Sources
Other Literature Sources
Research Materials