Modularly Programmable Nanoparticle Vaccine Based on Polyethyleneimine for Personalized Cancer Immunotherapy
- PMID: 33717838
- PMCID: PMC7927624
- DOI: 10.1002/advs.202002577
Modularly Programmable Nanoparticle Vaccine Based on Polyethyleneimine for Personalized Cancer Immunotherapy
Abstract
Nanoparticles (NPs) can serve as a promising vaccine delivery platform for improving pharmacological property and codelivery of antigens and adjuvants. However, NP-based vaccines are generally associated with complex synthesis and postmodification procedures, which pose technical and manufacturing challenges for tailor-made vaccine production. Here, modularly programmed, polyethyleneimine (PEI)-based NP vaccines are reported for simple production of personalized cancer vaccines. Briefly, PEI is conjugated with neoantigens by facile coupling chemistry, followed by electrostatic assembly with CpG adjuvants, leading to the self-assembly of nontoxic, sub-50 nm PEI NPs. Importantly, PEI NPs promote activation and antigen cross-presentation of antigen-presenting cells and cross-priming of neoantigen-specific CD8+ T cells. Surprisingly, after only a single intratumoral injection, PEI NPs with optimal PEGylation elicit as high as ≈30% neoantigen-specific CD8+ T cell response in the systemic circulation and sustain elevated CD8+ T cell response over 3 weeks. PEI-based nanovaccines exert potent antitumor efficacy against pre-established local tumors as well as highly aggressive metastatic tumors. PEI engineering for modular incorporation of neoantigens and adjuvants offers a promising strategy for rapid and facile production of personalized cancer vaccines.
Keywords: cancer vaccines; immunotherapy; nanoparticles; neoantigens.
© 2021 The Authors. Advanced Science published by Wiley‐VCH GmbH.
Conflict of interest statement
The authors declare no conflict of interest.
Figures
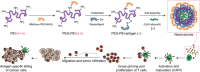
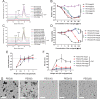
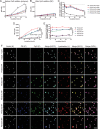
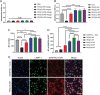
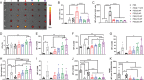
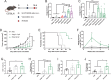
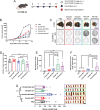
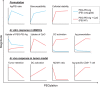
Similar articles
-
Personalized combination nano-immunotherapy for robust induction and tumor infiltration of CD8+ T cells.Biomaterials. 2021 Jul;274:120844. doi: 10.1016/j.biomaterials.2021.120844. Epub 2021 Apr 27. Biomaterials. 2021. PMID: 33962217 Free PMC article.
-
Responsive Multivesicular Polymeric Nanovaccines that Codeliver STING Agonists and Neoantigens for Combination Tumor Immunotherapy.Adv Sci (Weinh). 2022 Aug;9(23):e2201895. doi: 10.1002/advs.202201895. Epub 2022 Jun 16. Adv Sci (Weinh). 2022. PMID: 35712773 Free PMC article.
-
Self-assembly nanovaccine containing TLR7/8 agonist and STAT3 inhibitor enhances tumor immunotherapy by augmenting tumor-specific immune response.J Immunother Cancer. 2021 Aug;9(8):e003132. doi: 10.1136/jitc-2021-003132. J Immunother Cancer. 2021. PMID: 34452929 Free PMC article.
-
Nanovaccines for cancer immunotherapy: Focusing on complex formation between adjuvant and antigen.Int Immunopharmacol. 2023 Apr;117:109887. doi: 10.1016/j.intimp.2023.109887. Epub 2023 Feb 24. Int Immunopharmacol. 2023. PMID: 36841155 Review.
-
Engineering neoantigen vaccines to improve cancer personalized immunotherapy.Int J Biol Sci. 2022 Sep 1;18(15):5607-5623. doi: 10.7150/ijbs.76281. eCollection 2022. Int J Biol Sci. 2022. PMID: 36263174 Free PMC article. Review.
Cited by
-
Personalized combination nano-immunotherapy for robust induction and tumor infiltration of CD8+ T cells.Biomaterials. 2021 Jul;274:120844. doi: 10.1016/j.biomaterials.2021.120844. Epub 2021 Apr 27. Biomaterials. 2021. PMID: 33962217 Free PMC article.
-
Nanoplatform Based Intranasal Vaccines: Current Progress and Clinical Challenges.ACS Nano. 2024 Sep 10;18(36):24650-24681. doi: 10.1021/acsnano.3c10797. Epub 2024 Aug 26. ACS Nano. 2024. PMID: 39185745 Free PMC article. Review.
-
Bioorthogonal CRISPR/Cas9-Drug Conjugate: A Combinatorial Nanomedicine Platform.Adv Sci (Weinh). 2023 Sep;10(27):e2302253. doi: 10.1002/advs.202302253. Epub 2023 Jul 23. Adv Sci (Weinh). 2023. PMID: 37485817 Free PMC article.
-
Immune-regulating camouflaged nanoplatforms: A promising strategy to improve cancer nano-immunotherapy.Bioact Mater. 2022 Aug 10;21:1-19. doi: 10.1016/j.bioactmat.2022.07.023. eCollection 2023 Mar. Bioact Mater. 2022. PMID: 36017071 Free PMC article. Review.
-
Progress in reeducating tumor-associated macrophages in tumor microenvironment.Discov Oncol. 2024 Jul 26;15(1):312. doi: 10.1007/s12672-024-01186-8. Discov Oncol. 2024. PMID: 39060648 Free PMC article. Review.
References
-
- van der Burg S. H., Arens R., Ossendorp F., van Hall T., Melief C. J. M., Nat. Rev. Cancer 2016, 16, 219. - PubMed
-
- a) Kreiter S., Vormehr M., van de Roemer N., Diken M., Löwer M., Diekmann J., Boegel S., Schrörs B., Vascotto F., Castle J. C., Tadmor A. D., Schoenberger S. P., Huber C., Türeci Ö., Sahin U., Nature 2015, 520, 692; - PMC - PubMed
- b) Yadav M., Jhunjhunwala S., Phung Q. T., Lupardus P., Tanguay J., Bumbaca S., Franci C., Cheung T. K., Fritsche J., Weinschenk T., Modrusan Z., Mellman I., Lill J. R., Delamarre L., Nature 2014, 515, 572. - PubMed
-
- a) Ott P. A., Hu Z., Keskin D. B., Shukla S. A., Sun J., Bozym D. J., Zhang W., Luoma A., Giobbie‐Hurder A., Peter L., Chen C., Olive O., Carter T. A., Li S., Lieb D. J., Eisenhaure T., Gjini E., Stevens J., Lane W. J., Javeri I., Nellaiappan K., Salazar A. M., Daley H., Seaman M., Buchbinder E. I., Yoon C. H., Harden M., Lennon N., Gabriel S., Rodig S. J., Barouch D. H., Aster J. C., Getz G., Wucherpfennig K., Neuberg D., Ritz J., Lander E. S., Fritsch E. F., Hacohen N., Wu C. J., Nature 2017, 547, 217; - PMC - PubMed
- b) Sahin U., Derhovanessian E., Miller M., Kloke B.‐P., Simon P., Löwer M., Bukur V., Tadmor A. D., Luxemburger U., Schrörs B., Omokoko T., Vormehr M., Albrecht C., Paruzynski A., Kuhn A. N., Buck J., Heesch S., Schreeb K. H., Müller F., Ortseifer I., Vogler I., Godehardt E., Attig S., Rae R., Breitkreuz A., Tolliver C., Suchan M., Martic G., Hohberger A., Sorn P., Diekmann J., Ciesla J., Waksmann O., Brück A.‐K., Witt M., Zillgen M., Rothermel A., Kasemann B., Langer D., Bolte S., Diken M., Kreiter S., Nemecek R., Gebhardt C., Grabbe S., Höller C., Utikal J., Huber C., Loquai C., Türeci Ö., Nature 2017, 547, 222; - PubMed
- c) Hilf N., Kuttruff‐Coqui S., Frenzel K., Bukur V., Stevanović S., Gouttefangeas C., Platten M., Tabatabai G., Dutoit V., van der Burg S. H., thor Straten P., Martínez‐Ricarte F., Ponsati B., Okada H., Lassen U., Admon A., Ottensmeier C. H., Ulges A., Kreiter S., von Deimling A., Skardelly M., Migliorini D., Kroep J. R., Idorn M., Rodon J., Piró J., Poulsen H. S., Shraibman B., McCann K., Mendrzyk R., Löwer M., Stieglbauer M., Britten C. M., Capper D., Welters M. J. P., Sahuquillo J., Kiesel K., Derhovanessian E., Rusch E., Bunse L., Song C., Heesch S., Wagner C., Kemmer‐Brück A., Ludwig J., Castle J. C., Schoor O., Tadmor A. D., Green E., Fritsche J., Meyer M., Pawlowski N., Dorner S., Hoffgaard F., Rössler B., Maurer D., Weinschenk T., Reinhardt C., Huber C., Rammensee H.‐G., Singh‐Jasuja H., Sahin U., Dietrich P.‐Y., Wick W., Nature 2019, 565, 240; - PubMed
- d) Keskin D. B., Anandappa A. J., Sun J., Tirosh I., Mathewson N. D., Li S., Oliveira G., Giobbie‐Hurder A., Felt K., Gjini E., Shukla S. A., Hu Z., Li L., Le P. M., Allesøe R. L., Richman A. R., Kowalczyk M. S., Abdelrahman S., Geduldig J. E., Charbonneau S., Pelton K., Iorgulescu J. B., Elagina L., Zhang W., Olive O., McCluskey C., Olsen L. R., Stevens J., Lane W. J., Salazar A. M., Daley H., Wen P. Y., Chiocca E. A., Harden M., Lennon N. J., Gabriel S., Getz G., Lander E. S., Regev A., Ritz J., Neuberg D., Rodig S. J., Ligon K. L., Suvà M. L., Wucherpfennig K. W., Hacohen N., Fritsch E. F., Livak K. J., Ott P. A., Wu C. J., Reardon D. A., Nature 2019, 565, 234. - PMC - PubMed
-
- a) Liu H., Moynihan K. D., Zheng Y., Szeto G. L., Li A. V., Huang B., Van Egeren D. S., Park C., Irvine D. J., Nature 2014, 507, 519; - PMC - PubMed
- b) Zhu G., Lynn G. M., Jacobson O., Chen K., Liu Y., Zhang H., Ma Y., Zhang F., Tian R., Ni Q., Cheng S., Wang Z., Lu N., Yung B. C., Wang Z., Lang L., Fu X., Jin A., Weiss I. D., Vishwasrao H., Niu G., Shroff H., Klinman D. M., Seder R. A., Chen X., Nat. Commun. 2017, 8, 1954. - PMC - PubMed
Grants and funding
LinkOut - more resources
Full Text Sources
Other Literature Sources
Research Materials
Miscellaneous