The envelope protein of SARS-CoV-2 increases intra-Golgi pH and forms a cation channel that is regulated by pH
- PMID: 33709461
- PMCID: PMC8251088
- DOI: 10.1113/JP281037
The envelope protein of SARS-CoV-2 increases intra-Golgi pH and forms a cation channel that is regulated by pH
Abstract
Key points: We report a novel method for the transient expression of SARS-CoV-2 envelope (E) protein in intracellular organelles and the plasma membrane of mammalian cells and Xenopus oocytes. Intracellular expression of SARS-CoV-2 E protein increases intra-Golgi pH. By targeting the SARS-CoV-2 E protein to the plasma membrane, we show that it forms a cation channel, viroporin, that is modulated by changes of pH. This method for studying the activity of viroporins may facilitate screening for new antiviral drugs to identify novel treatments for COVID-19.
Abstract: The envelope (E) protein of coronaviruses such as SARS-CoV-1 is proposed to form an ion channel or viroporin that participates in viral propagation and pathogenesis. Here we developed a technique to study the E protein of SARS-CoV-2 in mammalian cells by directed targeting using a carboxyl-terminal fluorescent protein tag, mKate2. The wild-type SARS-CoV-2 E protein can be trafficked to intracellular organelles, notably the endoplasmic reticulum-Golgi intermediate complex, where its expression increases pH inside the organelle. We also succeeded in targeting SARS-CoV-2 E to the plasma membrane, which enabled biophysical analysis using whole-cell patch clamp recording in a mammalian cell line, HEK 293 cells, and two-electrode voltage clamp electrophysiology in Xenopus oocytes. The results suggest that the E protein forms an ion channel that is permeable to monovalent cations such as Na+ , Cs+ and K+ . The E current is nearly time- and voltage-independent when E protein is expressed in mammalian cells, and is modulated by changes of pH. At pH 6.0 and 7.4, the E protein current is activated, whereas at pH 8.0 and 9.0, the amplitude of E protein current is reduced, and in oocytes the inward E current fades at pH 9 in a time- and voltage-dependent manner. Using this directed targeting method and electrophysiological recordings, potential inhibitors of the E protein can be screened and subsequently investigated for antiviral activity against SARS-CoV-2 in vitro and possible efficacy in treating COVID-19.
Keywords: SARS-CoV-2; envelope protein; ion channel; membrane targeting.
© 2021 The Authors. The Journal of Physiology © 2021 The Physiological Society.
Figures
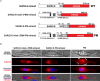
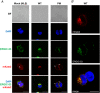
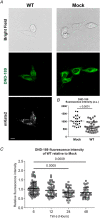
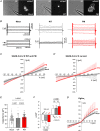
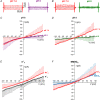
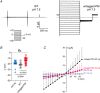
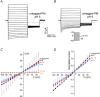
Comment in
-
SARS-CoV-2: pushing the E(nvelope).J Physiol. 2021 Jun;599(11):2785-2786. doi: 10.1113/JP281601. Epub 2021 May 8. J Physiol. 2021. PMID: 33873242 Free PMC article. No abstract available.
-
The role of the SARS-CoV-2 envelope protein as a pH-dependent cation channel.J Physiol. 2021 Jul;599(14):3435-3436. doi: 10.1113/JP281785. Epub 2021 Jun 24. J Physiol. 2021. PMID: 34089533 Free PMC article. No abstract available.
Similar articles
-
Patch-clamp studies and cell viability assays suggest a distinct site for viroporin inhibitors on the E protein of SARS-CoV-2.Virol J. 2023 Jul 8;20(1):142. doi: 10.1186/s12985-023-02095-y. Virol J. 2023. PMID: 37422646 Free PMC article.
-
Inhibition of SARS CoV Envelope Protein by Flavonoids and Classical Viroporin Inhibitors.Front Microbiol. 2021 Jul 8;12:692423. doi: 10.3389/fmicb.2021.692423. eCollection 2021. Front Microbiol. 2021. PMID: 34305855 Free PMC article.
-
SARS-CoV-2 infection alkalinizes the ERGIC and lysosomes through the viroporin activity of the viral envelope protein.J Cell Sci. 2023 Mar 15;136(6):jcs260685. doi: 10.1242/jcs.260685. Epub 2023 Mar 24. J Cell Sci. 2023. PMID: 36807531 Free PMC article.
-
Viroporins: Structure, function, and their role in the life cycle of SARS-CoV-2.Int J Biochem Cell Biol. 2022 Apr;145:106185. doi: 10.1016/j.biocel.2022.106185. Epub 2022 Feb 24. Int J Biochem Cell Biol. 2022. PMID: 35219876 Free PMC article. Review.
-
Might proton pump or sodium-hydrogen exchanger inhibitors be of value to ameliorate SARs-CoV-2 pathophysiology?Physiol Rep. 2021 Jan;8(24):e14649. doi: 10.14814/phy2.14649. Physiol Rep. 2021. PMID: 33369281 Free PMC article. Review.
Cited by
-
Potent NKT cell ligands overcome SARS-CoV-2 immune evasion to mitigate viral pathogenesis in mouse models.PLoS Pathog. 2023 Mar 24;19(3):e1011240. doi: 10.1371/journal.ppat.1011240. eCollection 2023 Mar. PLoS Pathog. 2023. PMID: 36961850 Free PMC article.
-
SARS-CoV2 Infection and the Importance of Potassium Balance.Front Med (Lausanne). 2021 Oct 27;8:744697. doi: 10.3389/fmed.2021.744697. eCollection 2021. Front Med (Lausanne). 2021. PMID: 34778307 Free PMC article.
-
Endogenous currents in HEK 293 cells are inhibited by memantine.Nat Chem Biol. 2023 Nov;19(11):1303-1305. doi: 10.1038/s41589-023-01423-1. Epub 2023 Oct 5. Nat Chem Biol. 2023. PMID: 37798356 No abstract available.
-
Electrophysiological properties and structural prediction of the SARS-CoV-2 viroprotein E.Front Mol Biosci. 2024 Mar 28;11:1334819. doi: 10.3389/fmolb.2024.1334819. eCollection 2024. Front Mol Biosci. 2024. PMID: 38606285 Free PMC article.
-
Reply to: How Many SARS-CoV-2 "Viroporins" Are Really Ion Channels?Commun Biol. 2022 Aug 25;5(1):860. doi: 10.1038/s42003-022-03670-9. Commun Biol. 2022. PMID: 36008476 Free PMC article. No abstract available.
References
-
- Arons MM, Hatfield KM, Reddy SC, Kimball A, James A, Jacobs JR, Taylor J, Spicer K, Bardossy AC, Oakley LP, Tanwar S, Dyal JW, Harney J, Chisty Z, Bell JM, Methner M, Paul P, Carlson CM, McLaughlin HP, Thornburg N, Tong S, Tamin A, Tao Y, Uehara A, Harcourt J, Clark S, Brostrom‐Smith C, Page LC, Kay M, Lewis J, Montgomery P, Stone ND, Clark TA, Honein MA, Duchin JS, Jernigan JA; Public Health–Seattle and King County and CDC COVID‐19 Investigation Team . (2020). Presymptomatic SARS‐CoV‐2 infections and transmission in a skilled nursing facility. N Engl J Med 382, 2081–2090. - PMC - PubMed
-
- Bedford T, Greninger AL, Roychoudhury P, Starita LM, Famulare M, Huang ML, Nalla A, Pepper G, Reinhardt A, Xie H, Shrestha L, Nguyen TN, Adler A, Brandstetter E, Cho S, Giroux D, Han PD, Fay K, Frazar CD, Ilcisin M, Lacombe K, Lee J, Kiavand A, Richardson M, Sibley TR, Truong M, Wolf CR, Nickerson DA, Rieder MJ, Englund JA; Seattle Flu Study Investigators , Hadfield J, Hodcroft EB, Huddleston J, Moncla LH, Müller NF, Neher RA, Deng X, Gu W, Federman S, Chiu C, Duchin JS, Gautom R, Melly G, Hiatt B, Dykema P, Lindquist S, Queen K, Tao Y, Uehara A, Tong S, MacCannell D, Armstrong GL, Baird GS, Chu HY, Shendure J & Jerome KR (2020). Cryptic transmission of SARS‐CoV‐2 in Washington state. Science 370, 571–575. - PMC - PubMed
Publication types
MeSH terms
Substances
Grants and funding
LinkOut - more resources
Full Text Sources
Other Literature Sources
Medical
Miscellaneous