The biology of thermoacidophilic archaea from the order Sulfolobales
- PMID: 33476388
- PMCID: PMC8557808
- DOI: 10.1093/femsre/fuaa063
The biology of thermoacidophilic archaea from the order Sulfolobales
Abstract
Thermoacidophilic archaea belonging to the order Sulfolobales thrive in extreme biotopes, such as sulfuric hot springs and ore deposits. These microorganisms have been model systems for understanding life in extreme environments, as well as for probing the evolution of both molecular genetic processes and central metabolic pathways. Thermoacidophiles, such as the Sulfolobales, use typical microbial responses to persist in hot acid (e.g. motility, stress response, biofilm formation), albeit with some unusual twists. They also exhibit unique physiological features, including iron and sulfur chemolithoautotrophy, that differentiate them from much of the microbial world. Although first discovered >50 years ago, it was not until recently that genome sequence data and facile genetic tools have been developed for species in the Sulfolobales. These advances have not only opened up ways to further probe novel features of these microbes but also paved the way for their potential biotechnological applications. Discussed here are the nuances of the thermoacidophilic lifestyle of the Sulfolobales, including their evolutionary placement, cell biology, survival strategies, genetic tools, metabolic processes and physiological attributes together with how these characteristics make thermoacidophiles ideal platforms for specialized industrial processes.
Keywords: Sulfolobales; Archaea; Thermoacidophiles.
© The Author(s) 2021. Published by Oxford University Press on behalf of FEMS. All rights reserved. For permissions, please e-mail: journals.permissions@oup.com.
Figures
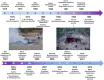
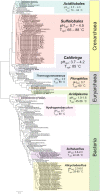
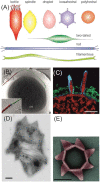
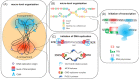
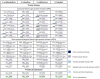
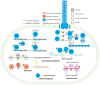
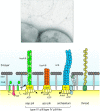
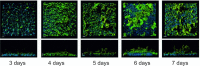
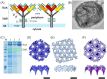
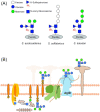
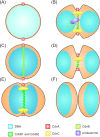
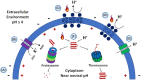
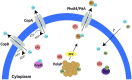
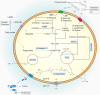
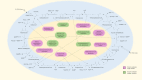
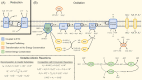
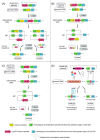
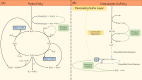
Similar articles
-
Extremely thermoacidophilic archaea for metal bioleaching: What do their genomes tell Us?Bioresour Technol. 2024 Jan;391(Pt B):129988. doi: 10.1016/j.biortech.2023.129988. Epub 2023 Nov 9. Bioresour Technol. 2024. PMID: 37949149 Review.
-
Genome analysis of the thermoacidophilic archaeon Acidianus copahuensis focusing on the metabolisms associated to biomining activities.BMC Genomics. 2017 Jun 6;18(1):445. doi: 10.1186/s12864-017-3828-x. BMC Genomics. 2017. PMID: 28587624 Free PMC article.
-
Stay or Go: Sulfolobales Biofilm Dispersal Is Dependent on a Bifunctional VapB Antitoxin.mBio. 2023 Apr 25;14(2):e0005323. doi: 10.1128/mbio.00053-23. Epub 2023 Apr 10. mBio. 2023. PMID: 37036347 Free PMC article.
-
Chalcopyrite bioleaching efficacy by extremely thermoacidophilic archaea leverages balanced iron and sulfur biooxidation.Bioresour Technol. 2024 Sep;408:131198. doi: 10.1016/j.biortech.2024.131198. Epub 2024 Aug 6. Bioresour Technol. 2024. PMID: 39097239
-
Physiology, Taxonomy, and Sulfur Metabolism of the Sulfolobales, an Order of Thermoacidophilic Archaea.Front Microbiol. 2021 Oct 14;12:768283. doi: 10.3389/fmicb.2021.768283. eCollection 2021. Front Microbiol. 2021. PMID: 34721370 Free PMC article. Review.
Cited by
-
Sulfide oxidation by members of the Sulfolobales.PNAS Nexus. 2024 May 23;3(6):pgae201. doi: 10.1093/pnasnexus/pgae201. eCollection 2024 Jun. PNAS Nexus. 2024. PMID: 38827816 Free PMC article.
-
Genomic Insights into the Ecological Role and Evolution of a Novel Thermoplasmata Order, "Candidatus Sysuiplasmatales".Appl Environ Microbiol. 2021 Oct 28;87(22):e0106521. doi: 10.1128/AEM.01065-21. Epub 2021 Sep 15. Appl Environ Microbiol. 2021. PMID: 34524897 Free PMC article.
-
Development of a defined medium for the heterotrophic cultivation of Metallosphaera sedula.Extremophiles. 2024 Jul 26;28(3):36. doi: 10.1007/s00792-024-01348-0. Extremophiles. 2024. PMID: 39060419 Free PMC article.
-
Utilization of formic acid by extremely thermoacidophilic archaea species.Microb Biotechnol. 2024 Sep;17(9):e70003. doi: 10.1111/1751-7915.70003. Microb Biotechnol. 2024. PMID: 39215388 Free PMC article.
-
Extremophiles in a changing world.Extremophiles. 2024 Apr 29;28(2):26. doi: 10.1007/s00792-024-01341-7. Extremophiles. 2024. PMID: 38683238 Free PMC article. Review.
References
-
- Aagaard C, Leviev I, Aravalli RN et al. General vectors for archaeal hyperthermophiles: strategies based on a mobile intron and a plasmid. FEMS Microbiol Rev. 1996;18:93–104. - PubMed
Publication types
MeSH terms
Substances
Grants and funding
LinkOut - more resources
Full Text Sources
Other Literature Sources