A Roadmap for the Molecular Farming of Viral Glycoprotein Vaccines: Engineering Glycosylation and Glycosylation-Directed Folding
- PMID: 33343609
- PMCID: PMC7744475
- DOI: 10.3389/fpls.2020.609207
A Roadmap for the Molecular Farming of Viral Glycoprotein Vaccines: Engineering Glycosylation and Glycosylation-Directed Folding
Abstract
Immunization with recombinant glycoprotein-based vaccines is a promising approach to induce protective immunity against viruses. However, the complex biosynthetic maturation requirements of these glycoproteins typically necessitate their production in mammalian cells to support their folding and post-translational modification. Despite these clear advantages, the incumbent costs and infrastructure requirements with this approach can be prohibitive in developing countries, and the production scales and timelines may prove limiting when applying these production systems to the control of pandemic viral outbreaks. Plant molecular farming of viral glycoproteins has been suggested as a cheap and rapidly scalable alternative production system, with the potential to perform post-translational modifications that are comparable to mammalian cells. Consequently, plant-produced glycoprotein vaccines for seasonal and pandemic influenza have shown promise in clinical trials, and vaccine candidates against the newly emergent severe acute respiratory syndrome coronavirus-2 have entered into late stage preclinical and clinical testing. However, many other viral glycoproteins accumulate poorly in plants, and are not appropriately processed along the secretory pathway due to differences in the host cellular machinery. Furthermore, plant-derived glycoproteins often contain glycoforms that are antigenically distinct from those present on the native virus, and may also be under-glycosylated in some instances. Recent advances in the field have increased the complexity and yields of biologics that can be produced in plants, and have now enabled the expression of many viral glycoproteins which could not previously be produced in plant systems. In contrast to the empirical optimization that predominated during the early years of molecular farming, the next generation of plant-made products are being produced by developing rational, tailor-made approaches to support their production. This has involved the elimination of plant-specific glycoforms and the introduction into plants of elements of the biosynthetic machinery from different expression hosts. These approaches have resulted in the production of mammalian N-linked glycans and the formation of O-glycan moieties in planta. More recently, plant molecular engineering approaches have also been applied to improve the glycan occupancy of proteins which are not appropriately glycosylated, and to support the folding and processing of viral glycoproteins where the cellular machinery differs from the usual expression host of the protein. Here we highlight recent achievements and remaining challenges in glycoengineering and the engineering of glycosylation-directed folding pathways in plants, and discuss how these can be applied to produce recombinant viral glycoproteins vaccines.
Keywords: calnexin; calreticulin; chaperones; folding; glycosylation; occupancy; oligosaccaryltransferase; processing.
Copyright © 2020 Margolin, Crispin, Meyers, Chapman and Rybicki.
Conflict of interest statement
EM, AM, and ER have filed a patent describing the co-expression of chaperone proteins to improve the production of heterologous polypeptides in plants. The remaning authors declare that the research was conducted in the absence of any commercial or financial relationships that could be construed as a potential conflict of interest.
Figures
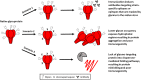
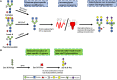
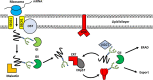
Similar articles
-
Site-Specific Glycosylation of Recombinant Viral Glycoproteins Produced in Nicotiana benthamiana.Front Plant Sci. 2021 Jul 22;12:709344. doi: 10.3389/fpls.2021.709344. eCollection 2021. Front Plant Sci. 2021. PMID: 34367227 Free PMC article.
-
Augmenting glycosylation-directed folding pathways enhances the fidelity of HIV Env immunogen production in plants.Biotechnol Bioeng. 2022 Oct;119(10):2919-2937. doi: 10.1002/bit.28169. Epub 2022 Jul 19. Biotechnol Bioeng. 2022. PMID: 35781691 Free PMC article.
-
Plant glycoengineering for designing next-generation vaccines and therapeutic proteins.Biotechnol Adv. 2023 Oct;67:108197. doi: 10.1016/j.biotechadv.2023.108197. Epub 2023 Jun 12. Biotechnol Adv. 2023. PMID: 37315875 Review.
-
A plant-produced SARS-CoV-2 spike protein elicits heterologous immunity in hamsters.Front Plant Sci. 2023 Mar 7;14:1146234. doi: 10.3389/fpls.2023.1146234. eCollection 2023. Front Plant Sci. 2023. PMID: 36959936 Free PMC article.
-
Production of complex viral glycoproteins in plants as vaccine immunogens.Plant Biotechnol J. 2018 Jun 11;16(9):1531-45. doi: 10.1111/pbi.12963. Online ahead of print. Plant Biotechnol J. 2018. PMID: 29890031 Free PMC article. Review.
Cited by
-
Harnessing Plant Sugar Metabolism for Glycoengineering.Biology (Basel). 2023 Dec 8;12(12):1505. doi: 10.3390/biology12121505. Biology (Basel). 2023. PMID: 38132331 Free PMC article.
-
Generation of multi-layered protein bodies in N. benthamiana for the encapsulation of vaccine antigens.Front Plant Sci. 2023 Jan 17;14:1109270. doi: 10.3389/fpls.2023.1109270. eCollection 2023. Front Plant Sci. 2023. PMID: 36733717 Free PMC article.
-
Site-Specific Glycosylation of Recombinant Viral Glycoproteins Produced in Nicotiana benthamiana.Front Plant Sci. 2021 Jul 22;12:709344. doi: 10.3389/fpls.2021.709344. eCollection 2021. Front Plant Sci. 2021. PMID: 34367227 Free PMC article.
-
Virus-like Particles: Fundamentals and Biomedical Applications.Int J Mol Sci. 2022 Aug 2;23(15):8579. doi: 10.3390/ijms23158579. Int J Mol Sci. 2022. PMID: 35955711 Free PMC article. Review.
-
Development of Plant-Based Vaccines for Prevention of Avian Influenza and Newcastle Disease in Poultry.Vaccines (Basel). 2022 Mar 19;10(3):478. doi: 10.3390/vaccines10030478. Vaccines (Basel). 2022. PMID: 35335110 Free PMC article. Review.
References
Publication types
Grants and funding
LinkOut - more resources
Full Text Sources
Research Materials