Aqueous outflow regulation - 21st century concepts
- PMID: 33217556
- PMCID: PMC8126645
- DOI: 10.1016/j.preteyeres.2020.100917
Aqueous outflow regulation - 21st century concepts
Abstract
We propose an integrated model of aqueous outflow control that employs a pump-conduit system in this article. Our model exploits accepted physiologic regulatory mechanisms such as those of the arterial, venous, and lymphatic systems. Here, we also provide a framework for developing novel diagnostic and therapeutic strategies to improve glaucoma patient care. In the model, the trabecular meshwork distends and recoils in response to continuous physiologic IOP transients like the ocular pulse, blinking, and eye movement. The elasticity of the trabecular meshwork determines cyclic volume changes in Schlemm's canal (SC). Tube-like SC inlet valves provide aqueous entry into the canal, and outlet valve leaflets at collector channels control aqueous exit from SC. Connections between the pressure-sensing trabecular meshwork and the outlet valve leaflets dynamically control flow from SC. Normal function requires regulation of the trabecular meshwork properties that determine distention and recoil. The aqueous pump-conduit provides short-term pressure control by varying stroke volume in response to pressure changes. Modulating TM constituents that regulate stroke volume provides long-term control. The aqueous outflow pump fails in glaucoma due to the loss of trabecular tissue elastance, as well as alterations in ciliary body tension. These processes lead to SC wall apposition and loss of motion. Visible evidence of pump failure includes a lack of pulsatile aqueous discharge into aqueous veins and reduced ability to reflux blood into SC. These alterations in the functional properties are challenging to monitor clinically. Phase-sensitive OCT now permits noninvasive, quantitative measurement of pulse-dependent TM motion in humans. This proposed conceptual model and related techniques offer a novel framework for understanding mechanisms, improving management, and development of therapeutic options for glaucoma.
Keywords: Aqueous outflow pump; Elastance; Glaucoma; Intraocular pressure regulation; Pulsatile aqueous outflow; Schlemm's canal valves.
Copyright © 2020 The Author(s). Published by Elsevier Ltd.. All rights reserved.
Figures
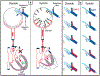
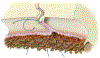
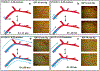
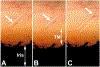
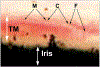
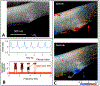
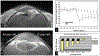
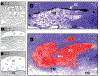
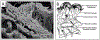
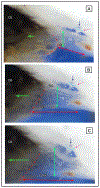
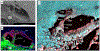
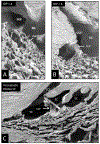
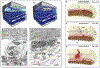
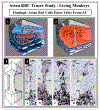
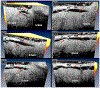
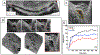
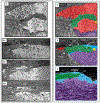
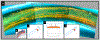
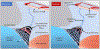
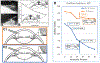
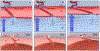
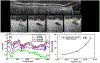
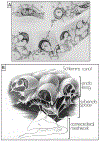
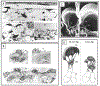
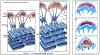
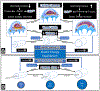
Similar articles
-
Aqueous outflow regulation: Optical coherence tomography implicates pressure-dependent tissue motion.Exp Eye Res. 2017 May;158:171-186. doi: 10.1016/j.exer.2016.06.007. Epub 2016 Jun 11. Exp Eye Res. 2017. PMID: 27302601 Free PMC article. Review.
-
Aqueous outflow - A continuum from trabecular meshwork to episcleral veins.Prog Retin Eye Res. 2017 Mar;57:108-133. doi: 10.1016/j.preteyeres.2016.12.004. Epub 2016 Dec 24. Prog Retin Eye Res. 2017. PMID: 28028002 Free PMC article. Review.
-
Pulsatile flow into the aqueous veins: manifestations in normal and glaucomatous eyes.Exp Eye Res. 2011 May;92(5):318-27. doi: 10.1016/j.exer.2011.03.011. Epub 2011 Mar 31. Exp Eye Res. 2011. PMID: 21440541 Free PMC article. Review.
-
Trabecular Meshwork Movement Controls Distal Valves and Chambers: New Glaucoma Medical and Surgical Targets.J Clin Med. 2023 Oct 18;12(20):6599. doi: 10.3390/jcm12206599. J Clin Med. 2023. PMID: 37892736 Free PMC article. Review.
-
Schlemm's canal: the outflow 'vessel'.Acta Ophthalmol. 2022 Jun;100(4):e881-e890. doi: 10.1111/aos.15027. Epub 2021 Sep 13. Acta Ophthalmol. 2022. PMID: 34519170 Free PMC article. Review.
Cited by
-
Long-term efficacy and safety of ab externo canaloplasty in the Polish Caucasian population with open-angle glaucoma: A 3-year retrospective study.PLoS One. 2024 Oct 16;19(10):e0312236. doi: 10.1371/journal.pone.0312236. eCollection 2024. PLoS One. 2024. PMID: 39413111 Free PMC article.
-
Historical and Contemporary Debates in Schlemm's Canal-Based MIGS.J Clin Med. 2024 Aug 19;13(16):4882. doi: 10.3390/jcm13164882. J Clin Med. 2024. PMID: 39201024 Free PMC article. Review.
-
Recent advances in optical coherence tomography for anterior segment imaging in small animals and their clinical implications.Ocul Surf. 2022 Oct;26:222-233. doi: 10.1016/j.jtos.2022.08.011. Epub 2022 Oct 3. Ocul Surf. 2022. PMID: 36195237 Free PMC article. Review.
-
Integrin Crosstalk and Its Effect on the Biological Functions of the Trabecular Meshwork/Schlemm's Canal.Front Cell Dev Biol. 2022 Apr 29;10:886702. doi: 10.3389/fcell.2022.886702. eCollection 2022. Front Cell Dev Biol. 2022. PMID: 35573686 Free PMC article. Review.
-
Inhibitory and Agonistic Autoantibodies Directed Against the β2-Adrenergic Receptor in Pseudoexfoliation Syndrome and Glaucoma.Front Neurosci. 2021 Aug 6;15:676579. doi: 10.3389/fnins.2021.676579. eCollection 2021. Front Neurosci. 2021. PMID: 34421514 Free PMC article.
References
-
- Alberts B, 2002. The cytoskeleton. In: Alberts B, Johnson A, Lewis J, Raff M, Roberts K, Walter P (Eds.), Molecular Biology of the Cell. Garland Science, New York.
-
- Alenghat FJ, Ingber DE, 2002. Mechanotransduction: all signals point to cytoskeleton, matrix, and integrins. Sci. STKE, 2002, pe6. - PubMed
-
- Allingham RR, Damji KF, Freedman SF, Moroi SE, Rhee DJ, Shields MB, 2012. Shields Textbook of Glaucoma. Lippincott Williams & Wilkins.
Publication types
MeSH terms
Grants and funding
LinkOut - more resources
Full Text Sources
Medical