Radiation Dose-Enhancement Is a Potent Radiotherapeutic Effect of Rare-Earth Composite Nanoscintillators in Preclinical Models of Glioblastoma
- PMID: 33101867
- PMCID: PMC7578894
- DOI: 10.1002/advs.202001675
Radiation Dose-Enhancement Is a Potent Radiotherapeutic Effect of Rare-Earth Composite Nanoscintillators in Preclinical Models of Glioblastoma
Abstract
To improve the prognosis of glioblastoma, innovative radiotherapy regimens are required to augment the effect of tolerable radiation doses while sparing surrounding tissues. In this context, nanoscintillators are emerging radiotherapeutics that down-convert X-rays into photons with energies ranging from UV to near-infrared. During radiotherapy, these scintillating properties amplify radiation-induced damage by UV-C emission or photodynamic effects. Additionally, nanoscintillators that contain high-Z elements are likely to induce another, currently unexplored effect: radiation dose-enhancement. This phenomenon stems from a higher photoelectric absorption of orthovoltage X-rays by high-Z elements compared to tissues, resulting in increased production of tissue-damaging photo- and Auger electrons. In this study, Geant4 simulations reveal that rare-earth composite LaF3:Ce nanoscintillators effectively generate photo- and Auger-electrons upon orthovoltage X-rays. 3D spatially resolved X-ray fluorescence microtomography shows that LaF3:Ce highly concentrates in microtumors and enhances radiotherapy in an X-ray energy-dependent manner. In an aggressive syngeneic model of orthotopic glioblastoma, intracerebral injection of LaF3:Ce is well tolerated and achieves complete tumor remission in 15% of the subjects receiving monochromatic synchrotron radiotherapy. This study provides unequivocal evidence for radiation dose-enhancement by nanoscintillators, eliciting a prominent radiotherapeutic effect. Altogether, nanoscintillators have invaluable properties for enhancing the focal damage of radiotherapy in glioblastoma and other radioresistant cancers.
Keywords: X‐ray‐induced photodynamic therapy; glioblastoma; nanoscintillators; radiation dose‐enhancement; synchrotron radiation.
© 2020 The Authors. Published by Wiley‐VCH GmbH.
Conflict of interest statement
The authors declare no conflict of interest.
Figures
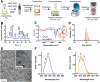
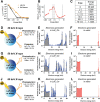
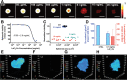
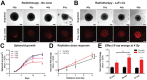
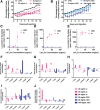
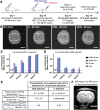
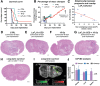
Similar articles
-
Modelling energy deposition in nanoscintillators to predict the efficiency of the X-ray-induced photodynamic effect.Nanoscale. 2015 Mar 19;7(13):5744-51. doi: 10.1039/c4nr07444k. Nanoscale. 2015. PMID: 25746211
-
Treatment plans optimization for contrast-enhanced synchrotron stereotactic radiotherapy.Med Phys. 2010 Jun;37(6):2445-56. doi: 10.1118/1.3327455. Med Phys. 2010. PMID: 20632555
-
Photoelectric-enhanced radiation therapy with quasi-monochromatic computed tomography.Med Phys. 2009 Jun;36(6):2107-17. doi: 10.1118/1.3125137. Med Phys. 2009. PMID: 19610300
-
Studies on the Exposure of Gadolinium Containing Nanoparticles with Monochromatic X-rays Drive Advances in Radiation Therapy.Nanomaterials (Basel). 2020 Jul 9;10(7):1341. doi: 10.3390/nano10071341. Nanomaterials (Basel). 2020. PMID: 32660093 Free PMC article. Review.
-
Nanoscintillators for microscopic diagnostics of biological and medical objects and medical therapy.IEEE Trans Nanobioscience. 2009 Mar;8(1):20-32. doi: 10.1109/TNB.2009.2016551. Epub 2009 Mar 16. IEEE Trans Nanobioscience. 2009. PMID: 19304500 Review.
Cited by
-
Lanthanum-based dendritic mesoporous nanoplatform for tumor microenvironment activating synergistic anti-glioma efficacy.Mater Today Bio. 2024 Sep 3;28:101223. doi: 10.1016/j.mtbio.2024.101223. eCollection 2024 Oct. Mater Today Bio. 2024. PMID: 39290466 Free PMC article.
-
Deep-Tissue Activation of Photonanomedicines: An Update and Clinical Perspectives.Cancers (Basel). 2022 Apr 15;14(8):2004. doi: 10.3390/cancers14082004. Cancers (Basel). 2022. PMID: 35454910 Free PMC article. Review.
-
Integrating Nanotechnology in Neurosurgery, Neuroradiology, and Neuro-Oncology Practice-The Clinicians' Perspective.Front Bioeng Biotechnol. 2022 Feb 9;10:801822. doi: 10.3389/fbioe.2022.801822. eCollection 2022. Front Bioeng Biotechnol. 2022. PMID: 35223783 Free PMC article. No abstract available.
-
Requirements for Designing an Effective Metallic Nanoparticle (NP)-Boosted Radiation Therapy (RT).Cancers (Basel). 2021 Jun 25;13(13):3185. doi: 10.3390/cancers13133185. Cancers (Basel). 2021. PMID: 34202342 Free PMC article. Review.
-
The Multifaceted Roles of Copper in Cancer: A Trace Metal Element with Dysregulated Metabolism, but Also a Target or a Bullet for Therapy.Cancers (Basel). 2020 Dec 1;12(12):3594. doi: 10.3390/cancers12123594. Cancers (Basel). 2020. PMID: 33271772 Free PMC article. Review.
References
-
- Stupp R., Mason W. P., van den Bent M. J., Weller M., Fisher B., Taphoorn M. J. B., Belanger K., Brandes A. A., Marosi C., Bogdahn U., Curschmann J., Janzer R. C., Ludwin S. K., Gorlia T., Allgeier A., Lacombe D., Cairncross J. G., Eisenhauer E., Mirimanoff R. O., N. Engl. J. Med. 2005, 352, 987. - PubMed
-
- Kagan A. R., Steckel R. J., Cancilla P., Juillard G., Patin T., Int. J. Radiat. Oncol., Biol., Phys. 1976, 1, 729. - PubMed
-
- Dujardin C., Auffray E., Bourret‐Courchesne E., Dorenbos P., Lecoq P., Nikl M., Vasil'ev A. N., Yoshikawa A., Zhu R., IEEE Trans. Nucl. Sci. 2018, 65, 1977.
-
- Chen X., Song J., Chen X., Yang H., Chem. Soc. Rev. 2019, 48, 3073. - PubMed
LinkOut - more resources
Full Text Sources