Microtubule-dependent pushing forces contribute to long-distance aster movement and centration in Xenopus laevis egg extracts
- PMID: 33026931
- PMCID: PMC7851858
- DOI: 10.1091/mbc.E20-01-0088
Microtubule-dependent pushing forces contribute to long-distance aster movement and centration in Xenopus laevis egg extracts
Abstract
During interphase of the eukaryotic cell cycle, the microtubule (MT) cytoskeleton serves as both a supportive scaffold for organelles and an arborized system of tracks for intracellular transport. At the onset of mitosis, the position of the astral MT network, specifically its center, determines the eventual location of the spindle apparatus and ultimately the cytokinetic furrow. Positioning of the MT aster often results in its movement to the center of a cell, even in large blastomeres hundreds of microns in diameter. This translocation requires positioning forces, yet how these forces are generated and then integrated within cells of various sizes and geometries remains an open question. Here we describe a method that combines microfluidics, hydrogels, and Xenopus laevis egg extract to investigate the mechanics of aster movement and centration. We determined that asters were able to find the center of artificial channels and annular cylinders, even when cytoplasmic dynein-dependent pulling mechanisms were inhibited. Characterization of aster movement away from V-shaped hydrogel barriers provided additional evidence for a MT-based pushing mechanism. Importantly, the distance over which this mechanism seemed to operate was longer than that predicted by radial aster growth models, agreeing with recent models of a more complex MT network architecture within the aster.
Figures
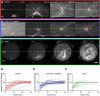
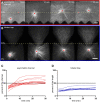
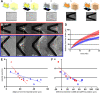
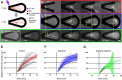
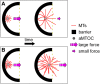
Similar articles
-
Co-movement of astral microtubules, organelles and F-actin by dynein and actomyosin forces in frog egg cytoplasm.Elife. 2020 Dec 7;9:e60047. doi: 10.7554/eLife.60047. Elife. 2020. PMID: 33284105 Free PMC article.
-
Branched microtubule nucleation and dynein transport organize RanGTP asters in Xenopus laevis egg extract.Mol Biol Cell. 2024 Jan 1;35(1):ar12. doi: 10.1091/mbc.E23-10-0407. Epub 2023 Nov 22. Mol Biol Cell. 2024. PMID: 37991893 Free PMC article.
-
Finding the cell center by a balance of dynein and myosin pulling and microtubule pushing: a computational study.Mol Biol Cell. 2010 Dec;21(24):4418-27. doi: 10.1091/mbc.E10-07-0627. Epub 2010 Oct 27. Mol Biol Cell. 2010. PMID: 20980619 Free PMC article.
-
Size Scaling of Microtubule Assemblies in Early Xenopus Embryos.Cold Spring Harb Perspect Biol. 2015 Aug 10;7(10):a019182. doi: 10.1101/cshperspect.a019182. Cold Spring Harb Perspect Biol. 2015. PMID: 26261283 Free PMC article. Review.
-
[Dynein and dynactin as organizers of the system of cell microtubules].Ontogenez. 2006 Sep-Oct;37(5):323-39. Ontogenez. 2006. PMID: 17066975 Review. Russian.
Cited by
-
Dynein-dependent collection of membranes defines the architecture and position of microtubule asters in isolated, geometrically confined volumes of cell-free extracts.Mol Biol Cell. 2022 Sep 15;33(11):br20. doi: 10.1091/mbc.E22-03-0074. Epub 2022 Aug 17. Mol Biol Cell. 2022. PMID: 35976715 Free PMC article.
-
The positioning mechanics of microtubule asters in Drosophila embryo explants.Elife. 2024 Mar 1;12:RP90541. doi: 10.7554/eLife.90541. Elife. 2024. PMID: 38426416 Free PMC article.
-
Building on-chip cytoskeletal circuits via branched microtubule networks.Proc Natl Acad Sci U S A. 2024 Jan 23;121(4):e2315992121. doi: 10.1073/pnas.2315992121. Epub 2024 Jan 17. Proc Natl Acad Sci U S A. 2024. PMID: 38232292 Free PMC article.
-
The Cytoskeleton and Its Roles in Self-Organization Phenomena: Insights from Xenopus Egg Extracts.Cells. 2021 Aug 26;10(9):2197. doi: 10.3390/cells10092197. Cells. 2021. PMID: 34571847 Free PMC article. Review.
-
Active condensation of filaments under spatial confinement.Front Phys. 2022;10:897255. doi: 10.3389/fphy.2022.897255. Epub 2022 Jun 24. Front Phys. 2022. PMID: 38116396 Free PMC article.
References
-
- Aist JR, Liang H, Berns MW (1993). Astral and spindle forces in PtK2 cells during anaphase B: a laser microbeam study. J Cell Sci (Pt 4), 1207–1216. - PubMed
Publication types
MeSH terms
Substances
Grants and funding
LinkOut - more resources
Full Text Sources