Dynamic elements of replication protein A at the crossroads of DNA replication, recombination, and repair
- PMID: 32856505
- PMCID: PMC7821911
- DOI: 10.1080/10409238.2020.1813070
Dynamic elements of replication protein A at the crossroads of DNA replication, recombination, and repair
Abstract
The heterotrimeric eukaryotic Replication protein A (RPA) is a master regulator of numerous DNA metabolic processes. For a long time, it has been viewed as an inert protector of ssDNA and a platform for assembly of various genome maintenance and signaling machines. Later, the modular organization of the RPA DNA binding domains suggested a possibility for dynamic interaction with ssDNA. This modular organization has inspired several models for the RPA-ssDNA interaction that aimed to explain how RPA, the high-affinity ssDNA binding protein, is replaced by the downstream players in DNA replication, recombination, and repair that bind ssDNA with much lower affinity. Recent studies, and in particular single-molecule observations of RPA-ssDNA interactions, led to the development of a new model for the ssDNA handoff from RPA to a specific downstream factor where not only stability and structural rearrangements but also RPA conformational dynamics guide the ssDNA handoff. Here we will review the current knowledge of the RPA structure, its dynamic interaction with ssDNA, and how RPA conformational dynamics may be influenced by posttranslational modification and proteins that interact with RPA, as well as how RPA dynamics may be harnessed in cellular decision making.
Keywords: Conformational protein dynamics; DNA repair; DNA replication; homologous recombination; protein-DNA interctions; replication protein A (RPA); single-molecule.
Figures
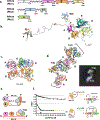
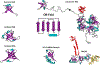
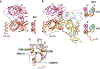
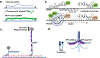
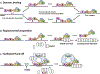
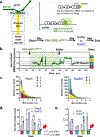
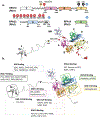
Similar articles
-
[Replication protein A as a major eukaryotic single-stranded DNA-binding protein and its role in DNA repair].Mol Biol (Mosk). 2016 Sep-Oct;50(5):735-750. doi: 10.7868/S0026898416030083. Mol Biol (Mosk). 2016. PMID: 27830676 Review. Russian.
-
Replication protein A: single-stranded DNA's first responder: dynamic DNA-interactions allow replication protein A to direct single-strand DNA intermediates into different pathways for synthesis or repair.Bioessays. 2014 Dec;36(12):1156-61. doi: 10.1002/bies.201400107. Epub 2014 Aug 29. Bioessays. 2014. PMID: 25171654 Free PMC article. Review.
-
Human replication protein A induces dynamic changes in single-stranded DNA and RNA structures.J Biol Chem. 2019 Sep 20;294(38):13915-13927. doi: 10.1074/jbc.RA119.009737. Epub 2019 Jul 26. J Biol Chem. 2019. PMID: 31350334 Free PMC article.
-
Replication Protein A, the Main Eukaryotic Single-Stranded DNA Binding Protein, a Focal Point in Cellular DNA Metabolism.Int J Mol Sci. 2024 Jan 2;25(1):588. doi: 10.3390/ijms25010588. Int J Mol Sci. 2024. PMID: 38203759 Free PMC article. Review.
-
Dynamic binding of replication protein a is required for DNA repair.Nucleic Acids Res. 2016 Jul 8;44(12):5758-72. doi: 10.1093/nar/gkw339. Epub 2016 Apr 29. Nucleic Acids Res. 2016. PMID: 27131385 Free PMC article.
Cited by
-
The human Shu complex promotes RAD51 activity by modulating RPA dynamics on ssDNA.Nat Commun. 2024 Aug 21;15(1):7197. doi: 10.1038/s41467-024-51595-0. Nat Commun. 2024. PMID: 39169038 Free PMC article.
-
A moving target for drug discovery: Structure activity relationship and many genome (de)stabilizing functions of the RAD52 protein.DNA Repair (Amst). 2022 Dec;120:103421. doi: 10.1016/j.dnarep.2022.103421. Epub 2022 Oct 27. DNA Repair (Amst). 2022. PMID: 36327799 Free PMC article. Review.
-
Harmonin homology domain-mediated interaction of RTEL1 helicase with RPA and DNA provides insights into its recruitment to DNA repair sites.Nucleic Acids Res. 2024 Feb 9;52(3):1450-1470. doi: 10.1093/nar/gkad1208. Nucleic Acids Res. 2024. PMID: 38153196 Free PMC article.
-
Human HELB is a processive motor protein that catalyzes RPA clearance from single-stranded DNA.Proc Natl Acad Sci U S A. 2022 Apr 12;119(15):e2112376119. doi: 10.1073/pnas.2112376119. Epub 2022 Apr 6. Proc Natl Acad Sci U S A. 2022. PMID: 35385349 Free PMC article.
-
Distinct RPA functions promote eukaryotic DNA replication initiation and elongation.Nucleic Acids Res. 2023 Oct 27;51(19):10506-10518. doi: 10.1093/nar/gkad765. Nucleic Acids Res. 2023. PMID: 37739410 Free PMC article.
References
-
- Wold MS (1997) Replication protein A: a heterotrimeric, single-stranded DNA-binding protein required for eukaryotic DNA metabolism. Annu Rev Biochem, 66, 61–92. - PubMed
-
- Iftode C, Daniely Y and Borowiec JA (1999) Replication protein A (RPA): the eukaryotic SSB. Crit Rev Biochem Mol Biol, 34, 141–180. - PubMed
-
- Brill SJ and Stillman B (1989) Yeast replication factor-A functions in the unwinding of the SV40 origin of DNA replication. Nature, 342, 92–95. - PubMed
-
- Nasheuer HP, von Winkler D, Schneider C, Dornreiter I, Gilbert I and Fanning E (1992) Purification and functional characterization of bovine RP-A in an in vitro SV40 DNA replication system. Chromosoma, 102, S52–59. - PubMed
Publication types
MeSH terms
Substances
Grants and funding
LinkOut - more resources
Full Text Sources
Molecular Biology Databases