Deconstructing Mechanisms of Diet-Microbiome-Immune Interactions
- PMID: 32814025
- PMCID: PMC7441819
- DOI: 10.1016/j.immuni.2020.07.015
Deconstructing Mechanisms of Diet-Microbiome-Immune Interactions
Abstract
Emerging evidence suggests that the effect of dietary intake on human health and disease is linked to both the immune system and the microbiota. Yet, we lack an integrated mechanistic model for how these three complex systems relate, limiting our ability to understand and treat chronic and infectious disease. Here, we review recent findings at the interface of microbiology, immunology, and nutrition, with an emphasis on experimentally tractable models and hypothesis-driven mechanistic work. We outline emerging mechanistic concepts and generalizable approaches to bridge the gap between microbial ecology and molecular mechanism. These set the stage for a new era of precision human nutrition informed by a deep and comprehensive knowledge of the diverse cell types in and on the human body.
Keywords: host receptors; immunology; metabolites; microbiome; nutrition.
Copyright © 2020 Elsevier Inc. All rights reserved.
Conflict of interest statement
Declaration of Interests The authors declare no competing interests.
Figures
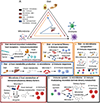
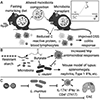
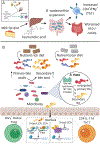
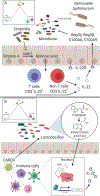
Similar articles
-
A review of metabolic potential of human gut microbiome in human nutrition.Arch Microbiol. 2018 Mar;200(2):203-217. doi: 10.1007/s00203-017-1459-x. Epub 2017 Nov 29. Arch Microbiol. 2018. PMID: 29188341 Review.
-
Deciphering the Chemical Lexicon of Host-Gut Microbiota Interactions.Trends Pharmacol Sci. 2019 Jun;40(6):430-445. doi: 10.1016/j.tips.2019.04.006. Epub 2019 May 9. Trends Pharmacol Sci. 2019. PMID: 31079848 Free PMC article. Review.
-
Molecules from the Microbiome.Annu Rev Biochem. 2021 Jun 20;90:789-815. doi: 10.1146/annurev-biochem-080320-115307. Epub 2021 Mar 26. Annu Rev Biochem. 2021. PMID: 33770448 Review.
-
Diet-microbiome interactions in cancer treatment: Opportunities and challenges for precision nutrition in cancer.Neoplasia. 2022 Jul;29:100800. doi: 10.1016/j.neo.2022.100800. Epub 2022 Apr 29. Neoplasia. 2022. PMID: 35500546 Free PMC article. Review.
-
The athletic gut microbiota.J Int Soc Sports Nutr. 2020 May 12;17(1):24. doi: 10.1186/s12970-020-00353-w. J Int Soc Sports Nutr. 2020. PMID: 32398103 Free PMC article. Review.
Cited by
-
The impact of the human gut microbiome on the treatment of autoimmune disease.Immunol Rev. 2024 Aug;325(1):107-130. doi: 10.1111/imr.13358. Epub 2024 Jun 12. Immunol Rev. 2024. PMID: 38864582 Review.
-
Diet-Regulating Microbiota and Host Immune System in Liver Disease.Int J Mol Sci. 2021 Jun 13;22(12):6326. doi: 10.3390/ijms22126326. Int J Mol Sci. 2021. PMID: 34199182 Free PMC article. Review.
-
Dietary non-starch polysaccharides impair immunity to enteric nematode infection.BMC Biol. 2023 Jun 14;21(1):138. doi: 10.1186/s12915-023-01640-z. BMC Biol. 2023. PMID: 37316905 Free PMC article.
-
Effects of altered salt intake and diet on cytokines in humans: A 20-week randomized cross-over intervention study.Eur J Immunol. 2023 Jan;53(1):e2250074. doi: 10.1002/eji.202250074. Epub 2022 Nov 20. Eur J Immunol. 2023. PMID: 36330564 Free PMC article. Clinical Trial.
-
Role of Ketogenic Diets in Multiple Sclerosis and Related Animal Models: An Updated Review.Adv Nutr. 2022 Oct 2;13(5):2002-2014. doi: 10.1093/advances/nmac065. Adv Nutr. 2022. PMID: 35679067 Free PMC article. Review.
References
-
- Ang QY, Alexander M, Newman JC, Tian Y, Cai J, Upadhyay V, Turnbaugh JA, Verdin E, Hall KD, Leibel LL, Ravussin E, Rosenbaum M, Patterson AD, and Turnbaugh PJ (2020). Ketogenesis alters the gut microbiome resulting in decreased intestinal Th17 levels. Cell, 10.1016/j.cell.2020.04.027. - DOI - PMC - PubMed
-
- Atarashi K, Tanoue T, Oshima K, Suda W, Nagano Y, Nishikawa H, Fukuda S, Saito T, Narushima S, Hase K, et al. (2013). Treg induction by a rationally selected mixture of Clostridia strains from the human microbiota. Nature 500, 232–236. - PubMed