Mechanisms and roles of mitochondrial localisation and dynamics in neuronal function
- PMID: 32714603
- PMCID: PMC7373250
- DOI: 10.1042/NS20200008
Mechanisms and roles of mitochondrial localisation and dynamics in neuronal function
Abstract
Neurons are highly polarised, complex and incredibly energy intensive cells, and their demand for ATP during neuronal transmission is primarily met by oxidative phosphorylation by mitochondria. Thus, maintaining the health and efficient function of mitochondria is vital for neuronal integrity, viability and synaptic activity. Mitochondria do not exist in isolation, but constantly undergo cycles of fusion and fission, and are actively transported around the neuron to sites of high energy demand. Intriguingly, axonal and dendritic mitochondria exhibit different morphologies. In axons mitochondria are small and sparse whereas in dendrites they are larger and more densely packed. The transport mechanisms and mitochondrial dynamics that underlie these differences, and their functional implications, have been the focus of concerted investigation. Moreover, it is now clear that deficiencies in mitochondrial dynamics can be a primary factor in many neurodegenerative diseases. Here, we review the role that mitochondrial dynamics play in neuronal function, how these processes support synaptic transmission and how mitochondrial dysfunction is implicated in neurodegenerative disease.
Keywords: DRP1; fission; fusion; mitochondria; mitochondrial dynamics; post translational modification.
© 2020 The Author(s).
Conflict of interest statement
The authors declare that there are no competing interests associated with the manuscript.
Figures
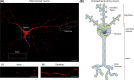
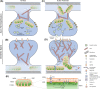
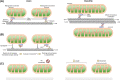
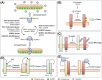
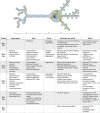
Similar articles
-
Chronic Energy Depletion due to Iron Deficiency Impairs Dendritic Mitochondrial Motility during Hippocampal Neuron Development.J Neurosci. 2019 Jan 30;39(5):802-813. doi: 10.1523/JNEUROSCI.1504-18.2018. Epub 2018 Dec 6. J Neurosci. 2019. PMID: 30523068 Free PMC article.
-
Mitochondrial dynamics in neuronal injury, development and plasticity.J Cell Sci. 2017 Feb 15;130(4):671-681. doi: 10.1242/jcs.171017. Epub 2017 Feb 2. J Cell Sci. 2017. PMID: 28154157 Free PMC article. Review.
-
Mitochondrial trafficking and morphology in neuronal injury.Biochim Biophys Acta. 2010 Jan;1802(1):143-50. doi: 10.1016/j.bbadis.2009.09.005. Epub 2009 Sep 10. Biochim Biophys Acta. 2010. PMID: 19747973 Review.
-
Dynamin-related protein 1: A critical protein in the pathogenesis of neural system dysfunctions and neurodegenerative diseases.J Cell Physiol. 2019 Jul;234(7):10032-10046. doi: 10.1002/jcp.27866. Epub 2018 Dec 4. J Cell Physiol. 2019. PMID: 30515821 Review.
-
Mitochondrial fusion/fission dynamics in neurodegeneration and neuronal plasticity.Neurobiol Dis. 2016 Jun;90:3-19. doi: 10.1016/j.nbd.2015.10.011. Epub 2015 Oct 19. Neurobiol Dis. 2016. PMID: 26494254 Review.
Cited by
-
Motor proteins at the mitochondria-cytoskeleton interface.J Cell Sci. 2021 Apr 1;134(7):jcs226084. doi: 10.1242/jcs.226084. Epub 2021 Apr 13. J Cell Sci. 2021. PMID: 33912943 Free PMC article. Review.
-
Novel therapeutic strategies targeting mitochondria as a gateway in neurodegeneration.Neural Regen Res. 2023 May;18(5):991-995. doi: 10.4103/1673-5374.355750. Neural Regen Res. 2023. PMID: 36254979 Free PMC article. Review.
-
Role of cyclin-dependent kinase 5 in early brain injury following experimental subarachnoid hemorrhage.Exp Ther Med. 2022 Feb;23(2):147. doi: 10.3892/etm.2021.11070. Epub 2021 Dec 15. Exp Ther Med. 2022. PMID: 35069828 Free PMC article.
-
AMPK activation attenuates cancer-induced bone pain by reducing mitochondrial dysfunction-mediated neuroinflammation.Acta Biochim Biophys Sin (Shanghai). 2023 Mar 25;55(3):460-471. doi: 10.3724/abbs.2023039. Acta Biochim Biophys Sin (Shanghai). 2023. PMID: 36971458 Free PMC article.
-
Mitochondrial Bioenergy in Neurodegenerative Disease: Huntington and Parkinson.Int J Mol Sci. 2023 Apr 13;24(8):7221. doi: 10.3390/ijms24087221. Int J Mol Sci. 2023. PMID: 37108382 Free PMC article. Review.
References
Publication types
LinkOut - more resources
Full Text Sources
Miscellaneous