Evolutionary Arms Race between Virus and Host Drives Genetic Diversity in Bat Severe Acute Respiratory Syndrome-Related Coronavirus Spike Genes
- PMID: 32699095
- PMCID: PMC7527062
- DOI: 10.1128/JVI.00902-20
Evolutionary Arms Race between Virus and Host Drives Genetic Diversity in Bat Severe Acute Respiratory Syndrome-Related Coronavirus Spike Genes
Abstract
The Chinese horseshoe bat (Rhinolophus sinicus), reservoir host of severe acute respiratory syndrome coronavirus (SARS-CoV), carries many bat SARS-related CoVs (SARSr-CoVs) with high genetic diversity, particularly in the spike gene. Despite these variations, some bat SARSr-CoVs can utilize the orthologs of the human SARS-CoV receptor, angiotensin-converting enzyme 2 (ACE2), for entry. It is speculated that the interaction between bat ACE2 and SARSr-CoV spike proteins drives diversity. Here, we identified a series of R. sinicus ACE2 variants with some polymorphic sites involved in the interaction with the SARS-CoV spike protein. Pseudoviruses or SARSr-CoVs carrying different spike proteins showed different infection efficiencies in cells transiently expressing bat ACE2 variants. Consistent results were observed by binding affinity assays between SARS-CoV and SARSr-CoV spike proteins and receptor molecules from bats and humans. All tested bat SARSr-CoV spike proteins had a higher binding affinity to human ACE2 than to bat ACE2, although they showed a 10-fold lower binding affinity to human ACE2 compared with that of their SARS-CoV counterpart. Structure modeling revealed that the difference in binding affinity between spike and ACE2 might be caused by the alteration of some key residues in the interface of these two molecules. Molecular evolution analysis indicates that some key residues were under positive selection. These results suggest that the SARSr-CoV spike protein and R. sinicus ACE2 may have coevolved over time and experienced selection pressure from each other, triggering the evolutionary arms race dynamics.IMPORTANCE Evolutionary arms race dynamics shape the diversity of viruses and their receptors. Identification of key residues which are involved in interspecies transmission is important to predict potential pathogen spillover from wildlife to humans. Previously, we have identified genetically diverse SARSr-CoVs in Chinese horseshoe bats. Here, we show the highly polymorphic ACE2 in Chinese horseshoe bat populations. These ACE2 variants support SARS-CoV and SARSr-CoV infection but with different binding affinities to different spike proteins. The higher binding affinity of SARSr-CoV spike to human ACE2 suggests that these viruses have the capacity for spillover to humans. The positive selection of residues at the interface between ACE2 and SARSr-CoV spike protein suggests long-term and ongoing coevolutionary dynamics between them. Continued surveillance of this group of viruses in bats is necessary for the prevention of the next SARS-like disease.
Keywords: ACE2; Chinese horseshoe bat; SARS-related coronavirus; genetic diversity; receptor; spike gene.
Copyright © 2020 American Society for Microbiology.
Figures
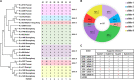
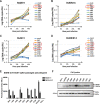
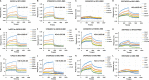
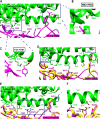
Similar articles
-
Epidemiology and Genomic Characterization of Two Novel SARS-Related Coronaviruses in Horseshoe Bats from Guangdong, China.mBio. 2022 Jun 28;13(3):e0046322. doi: 10.1128/mbio.00463-22. Epub 2022 Apr 25. mBio. 2022. PMID: 35467426 Free PMC article.
-
Severe Acute Respiratory Syndrome (SARS) Coronavirus ORF8 Protein Is Acquired from SARS-Related Coronavirus from Greater Horseshoe Bats through Recombination.J Virol. 2015 Oct;89(20):10532-47. doi: 10.1128/JVI.01048-15. Epub 2015 Aug 12. J Virol. 2015. PMID: 26269185 Free PMC article.
-
Isolation and characterization of a bat SARS-like coronavirus that uses the ACE2 receptor.Nature. 2013 Nov 28;503(7477):535-8. doi: 10.1038/nature12711. Epub 2013 Oct 30. Nature. 2013. PMID: 24172901 Free PMC article.
-
Molecular epidemiology, evolution and phylogeny of SARS coronavirus.Infect Genet Evol. 2019 Jul;71:21-30. doi: 10.1016/j.meegid.2019.03.001. Epub 2019 Mar 4. Infect Genet Evol. 2019. PMID: 30844511 Free PMC article. Review.
-
Angiotensin-converting enzyme 2: The old door for new severe acute respiratory syndrome coronavirus 2 infection.Rev Med Virol. 2020 Sep;30(5):e2122. doi: 10.1002/rmv.2122. Epub 2020 Jun 30. Rev Med Virol. 2020. PMID: 32602627 Free PMC article. Review.
Cited by
-
Structural characteristics of BtKY72 RBD bound to bat ACE2 reveal multiple key residues affecting ACE2 usage of sarbecoviruses.mBio. 2024 Sep 11;15(9):e0140424. doi: 10.1128/mbio.01404-24. Epub 2024 Jul 31. mBio. 2024. PMID: 39082798 Free PMC article.
-
Universal subunit vaccine protects against multiple SARS-CoV-2 variants and SARS-CoV.NPJ Vaccines. 2024 Jul 25;9(1):133. doi: 10.1038/s41541-024-00922-z. NPJ Vaccines. 2024. PMID: 39054338 Free PMC article.
-
Emergence of SARS and COVID-19 and preparedness for the next emerging disease X.Front Med. 2024 Feb;18(1):1-18. doi: 10.1007/s11684-024-1066-6. Epub 2024 Apr 2. Front Med. 2024. PMID: 38561562 Review.
-
ACE2-using merbecoviruses: Further evidence of convergent evolution of ACE2 recognition by NeoCoV and other MERS-CoV related viruses.Cell Insight. 2024 Jan 30;3(1):100145. doi: 10.1016/j.cellin.2023.100145. eCollection 2024 Feb. Cell Insight. 2024. PMID: 38476250 Free PMC article. Review.
-
Distinct phenotype of SARS-CoV-2 Omicron BA.1 in human primary cells but no increased host range in cell lines of putative mammalian reservoir species.Virus Res. 2024 Jan 2;339:199255. doi: 10.1016/j.virusres.2023.199255. Epub 2023 Nov 6. Virus Res. 2024. PMID: 38389324 Free PMC article.
References
-
- Woo PCY, Lau SKP, Lam CSF, Lau CCY, Tsang AKL, Lau JHN, Bai R, Teng JLL, Tsang CCC, Wang M, Zheng B-J, Chan K-H, Yuen K-Y. 2012. Discovery of seven novel mammalian and avian coronaviruses in the genus Deltacoronavirus supports bat coronaviruses as the gene source of Alphacoronavirus and Betacoronavirus and avian coronaviruses as the gene source of Gammacoronavirus and Deltacoronavirus. J Virol 86:3995–4008. doi:10.1128/JVI.06540-11. - DOI - PMC - PubMed
-
- Zhong NS, Zheng BJ, Li YM, Poon Xie ZH, Chan KH, Li PH, Tan SY, Chang Q, Xie JP, Liu XQ, Xu J, Li DX, Yuen KY, Peiris JSM, Guan Y. 2003. Epidemiology and cause of severe acute respiratory syndrome (SARS) in Guangdong, People’s Republic of China, in February, 2003. Lancet 362:1353–1358. doi:10.1016/S0140-6736(03)14630-2. - DOI - PMC - PubMed
-
- Drosten C, Gunther S, Preiser W, van der Werf S, Brodt HR, Becker S, Rabenau H, Panning M, Kolesnikova L, Fouchier RA, Berger A, Burguiere AM, Cinatl J, Eickmann M, Escriou N, Grywna K, Kramme S, Manuguerra JC, Muller S, Rickerts V, Sturmer M, Vieth S, Klenk HD, Osterhaus AD, Schmitz H, Doerr HW. 2003. Identification of a novel coronavirus in patients with severe acute respiratory syndrome. N Engl J Med 348:1967–1976. doi:10.1056/NEJMoa030747. - DOI - PubMed
-
- Ksiazek TG, Erdman D, Goldsmith CS, Zaki SR, Peret T, Emery S, Tong S, Urbani C, Comer JA, Lim W, Rollin PE, Dowell SF, Ling AE, Humphrey CD, Shieh WJ, Guarner J, Paddock CD, Rota P, Fields B, DeRisi J, Yang JY, Cox N, Hughes JM, LeDuc JW, Bellini WJ, Anderson LJ, Group SW, SARS Working Group. 2003. A novel coronavirus associated with severe acute respiratory syndrome. N Engl J Med 348:1953–1966. doi:10.1056/NEJMoa030781. - DOI - PubMed
Publication types
MeSH terms
Substances
LinkOut - more resources
Full Text Sources
Miscellaneous