Emerging Approaches to Functionalizing Cell Membrane-Coated Nanoparticles
- PMID: 32452667
- PMCID: PMC8507422
- DOI: 10.1021/acs.biochem.0c00343
Emerging Approaches to Functionalizing Cell Membrane-Coated Nanoparticles
Abstract
There has been significant interest in developing cell membrane-coated nanoparticles due to their unique abilities of biomimicry and biointerfacing. As the technology progresses, it becomes clear that the application of these nanoparticles can be drastically broadened if additional functions beyond those derived from the natural cell membranes can be integrated. Herein, we summarize the most recent advances in the functionalization of cell membrane-coated nanoparticles. In particular, we focus on emerging methods, including (1) lipid insertion, (2) membrane hybridization, (3) metabolic engineering, and (4) genetic modification. These approaches contribute diverse functions in a nondisruptive fashion while preserving the natural function of the cell membranes. They also improve on the multifunctional and multitasking ability of cell membrane-coated nanoparticles, making them more adaptive to the complexity of biological systems. We hope that these approaches will serve as inspiration for more strategies and innovations to advance cell membrane coating technology.
Figures
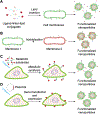
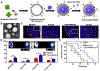
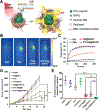
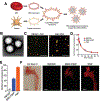
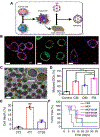
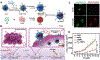
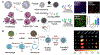
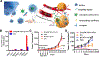
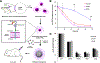
Similar articles
-
Advances in cell membrane-coated nanoparticles and their applications for bone therapy.Biomater Adv. 2023 Jan;144:213232. doi: 10.1016/j.bioadv.2022.213232. Epub 2022 Dec 5. Biomater Adv. 2023. PMID: 36502750 Review.
-
Cell membrane-coated nanoparticles: research advances.Nanomedicine (Lond). 2020 Mar;15(6):625-641. doi: 10.2217/nnm-2019-0388. Epub 2020 Feb 26. Nanomedicine (Lond). 2020. PMID: 32098564 Review.
-
Cell membrane-coated nanoparticles for targeting carcinogenic bacteria.Adv Drug Deliv Rev. 2024 Jun;209:115320. doi: 10.1016/j.addr.2024.115320. Epub 2024 Apr 21. Adv Drug Deliv Rev. 2024. PMID: 38643841 Review.
-
Cell membrane-camouflaged nanoparticles for drug delivery.J Control Release. 2015 Dec 28;220(Pt B):600-7. doi: 10.1016/j.jconrel.2015.07.019. Epub 2015 Jul 23. J Control Release. 2015. PMID: 26210440 Free PMC article. Review.
-
Cell membrane-coated nanoparticles: a novel multifunctional biomimetic drug delivery system.Drug Deliv Transl Res. 2023 Mar;13(3):716-737. doi: 10.1007/s13346-022-01252-0. Epub 2022 Nov 22. Drug Deliv Transl Res. 2023. PMID: 36417162 Free PMC article. Review.
Cited by
-
Biomimetic cell membrane-coated poly(lactic-co-glycolic acid) nanoparticles for biomedical applications.Bioeng Transl Med. 2022 Nov 2;8(2):e10441. doi: 10.1002/btm2.10441. eCollection 2023 Mar. Bioeng Transl Med. 2022. PMID: 36925703 Free PMC article. Review.
-
Recent Advances of Cell Membrane Coated Nanoparticles in Treating Cardiovascular Disorders.Molecules. 2021 Jun 5;26(11):3428. doi: 10.3390/molecules26113428. Molecules. 2021. PMID: 34198794 Free PMC article. Review.
-
Nanotechnology for virus treatment.Nano Today. 2021 Feb;36:101031. doi: 10.1016/j.nantod.2020.101031. Epub 2020 Dec 1. Nano Today. 2021. PMID: 33519948 Free PMC article. Review.
-
Research Advances of Cellular Nanoparticles as Multiplex Countermeasures.ACS Nano. 2024 Nov 5;18(44):30211-30223. doi: 10.1021/acsnano.4c09830. Epub 2024 Oct 23. ACS Nano. 2024. PMID: 39441568 Free PMC article. Review.
-
Cell Membrane-Coated Nanoparticles for Precision Medicine: A Comprehensive Review of Coating Techniques for Tissue-Specific Therapeutics.Int J Mol Sci. 2024 Feb 8;25(4):2071. doi: 10.3390/ijms25042071. Int J Mol Sci. 2024. PMID: 38396747 Free PMC article. Review.
References
-
- Ragelle H, Danhier F, Preat V, Langer R, and Anderson DG (2017) Nanoparticle-based drug delivery systems: a commercial and regulatory outlook as the field matures, Expert Opin. Drug Deliv 14, 851–864. - PubMed
-
- Piao JG, Wang LM, Gao F, You YZ, Xiong YJ, and Yang LH (2014) Erythrocyte Membrane Is an Alternative Coating to Polyethylene Glycol for Prolonging the Circulation Lifetime of Gold Nanocages for Photothermal Therapy, ACS Nano 8, 10414–10425. - PubMed