Effect of Aβ Oligomers on Neuronal APP Triggers a Vicious Cycle Leading to the Propagation of Synaptic Plasticity Alterations to Healthy Neurons
- PMID: 32444385
- PMCID: PMC7329309
- DOI: 10.1523/JNEUROSCI.2501-19.2020
Effect of Aβ Oligomers on Neuronal APP Triggers a Vicious Cycle Leading to the Propagation of Synaptic Plasticity Alterations to Healthy Neurons
Abstract
Alterations of excitatory synaptic function are the strongest correlate to the pathologic disturbance of cognitive ability observed in the early stages of Alzheimer's disease (AD). This pathologic feature is driven by amyloid-β oligomers (Aβos) and propagates from neuron to neuron. Here, we investigated the mechanism by which Aβos affect the function of synapses and how these alterations propagate to surrounding healthy neurons. We used complementary techniques ranging from electrophysiological recordings and molecular biology to confocal microscopy in primary cortical cultures, and from acute hippocampal and cortical slices from male wild-type and amyloid precursor protein (APP) knock-out (KO) mice to assess the effects of Aβos on glutamatergic transmission, synaptic plasticity, and dendritic spine structure. We showed that extracellular application of Aβos reduced glutamatergic synaptic transmission and long-term potentiation. These alterations were not observed in APP KO neurons, suggesting that APP expression is required. We demonstrated that Aβos/APP interaction increases the amyloidogenic processing of APP leading to intracellular accumulation of newly produced Aβos. Intracellular Aβos participate in synaptic dysfunctions as shown by pharmacological inhibition of APP processing or by intraneuronal infusion of an antibody raised against Aβos. Furthermore, we provide evidence that following APP processing, extracellular release of Aβos mediates the propagation of the synaptic pathology characterized by a decreased spine density of neighboring healthy neurons in an APP-dependent manner. Together, our data unveil a complementary role for Aβos in AD, while intracellular Aβos alter synaptic function, extracellular Aβos promote a vicious cycle that propagates synaptic pathology from diseased to healthy neurons.SIGNIFICANCE STATEMENT Here we provide the proof that a vicious cycle between extracellular and intracellular pools of Aβ oligomers (Aβos) is required for the spreading of Alzheimer's disease (AD) pathology. We showed that extracellular Aβos propagate excitatory synaptic alterations by promoting amyloid precursor protein (APP) processing. Our results also suggest that subsequent to APP cleavage two pools of Aβos are produced. One pool accumulates inside the cytosol, inducing the loss of synaptic plasticity potential. The other pool is released into the extracellular space and contributes to the propagation of the pathology from diseased to healthy neurons. Pharmacological strategies targeting the proteolytic cleavage of APP disrupt the relationship between extracellular and intracellular Aβ, providing a therapeutic approach for the disease.
Keywords: APP KO mice; APP processing; Alzheimer's disease; NMDA-dependent synaptic transmission; synaptic plasticity; β- and γ-secretase inhibition.
Copyright © 2020 the authors.
Figures
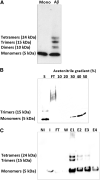
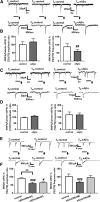
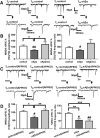
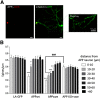
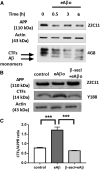
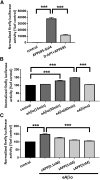
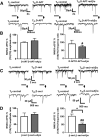
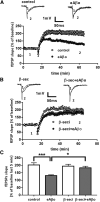
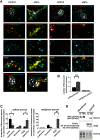
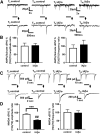
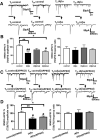
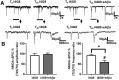
Similar articles
-
Human Brain-Derived Aβ Oligomers Bind to Synapses and Disrupt Synaptic Activity in a Manner That Requires APP.J Neurosci. 2017 Dec 6;37(49):11947-11966. doi: 10.1523/JNEUROSCI.2009-17.2017. Epub 2017 Nov 3. J Neurosci. 2017. PMID: 29101243 Free PMC article.
-
Intracellular accumulation of amyloid-β (Aβ) protein plays a major role in Aβ-induced alterations of glutamatergic synaptic transmission and plasticity.J Neurosci. 2014 Sep 17;34(38):12893-903. doi: 10.1523/JNEUROSCI.1201-14.2014. J Neurosci. 2014. PMID: 25232124 Free PMC article.
-
Synaptic activity reduces intraneuronal Abeta, promotes APP transport to synapses, and protects against Abeta-related synaptic alterations.J Neurosci. 2009 Aug 5;29(31):9704-13. doi: 10.1523/JNEUROSCI.2292-09.2009. J Neurosci. 2009. PMID: 19657023 Free PMC article.
-
Beta-amyloid, neuronal death and Alzheimer's disease.Curr Mol Med. 2001 Dec;1(6):733-7. doi: 10.2174/1566524013363177. Curr Mol Med. 2001. PMID: 11899259 Review.
-
Deregulation of excitatory neurotransmission underlying synapse failure in Alzheimer's disease.J Neurochem. 2013 Jul;126(2):191-202. doi: 10.1111/jnc.12304. Epub 2013 May 28. J Neurochem. 2013. PMID: 23668663 Review.
Cited by
-
Rational fusion design inspired by cell-penetrating peptide: SS31/S-14 G Humanin hybrid peptide with amplified multimodal efficacy and bio-permeability for the treatment of Alzheimer's disease.Asian J Pharm Sci. 2024 Aug;19(4):100938. doi: 10.1016/j.ajps.2024.100938. Epub 2024 Jun 24. Asian J Pharm Sci. 2024. PMID: 39253611 Free PMC article.
-
Insulin Resistance Exacerbates Alzheimer Disease via Multiple Mechanisms.Front Neurosci. 2021 Jul 19;15:687157. doi: 10.3389/fnins.2021.687157. eCollection 2021. Front Neurosci. 2021. PMID: 34349617 Free PMC article. Review.
-
Firing Alterations of Neurons in Alzheimer's Disease: Are They Merely a Consequence of Pathogenesis or a Pivotal Component of Disease Progression?Cells. 2024 Feb 29;13(5):434. doi: 10.3390/cells13050434. Cells. 2024. PMID: 38474398 Free PMC article. Review.
-
Long term worsening of amyloid pathology, cerebral function, and cognition after a single inoculation of beta-amyloid seeds with Osaka mutation.Acta Neuropathol Commun. 2023 Apr 22;11(1):66. doi: 10.1186/s40478-023-01559-0. Acta Neuropathol Commun. 2023. PMID: 37087498 Free PMC article.
-
Amyloid Precursor Protein: A Regulatory Hub in Alzheimer's Disease.Aging Dis. 2024 Feb 1;15(1):201-225. doi: 10.14336/AD.2023.0308. Aging Dis. 2024. PMID: 37307834 Free PMC article. Review.
References
Publication types
MeSH terms
Substances
LinkOut - more resources
Full Text Sources
Molecular Biology Databases
Research Materials