The physical basis and practical consequences of biological promiscuity
- PMID: 32244231
- PMCID: PMC9291633
- DOI: 10.1088/1478-3975/ab8697
The physical basis and practical consequences of biological promiscuity
Abstract
Proteins interact with metabolites, nucleic acids, and other proteins to orchestrate the myriad catalytic, structural and regulatory functions that support life from the simplest microbes to the most complex multicellular organisms. These molecular interactions are often exquisitely specific, but never perfectly so. Adventitious "promiscuous" interactions are ubiquitous due to the thousands of macromolecules and small molecules crowded together in cells. Such interactions may perturb protein function at the molecular level, but as long as they do not compromise organismal fitness, they will not be removed by natural selection. Although promiscuous interactions are physiologically irrelevant, they are important because they can provide a vast reservoir of potential functions that can provide the starting point for evolution of new functions, both in nature and in the laboratory.
Keywords: enzyme evolution; evolution; moonlighting; promiscuity; protein dynamics; protein-protein interaction.
© 2020 IOP Publishing Ltd.
Figures
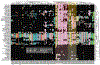
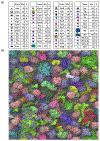
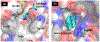
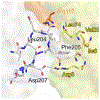
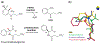
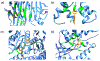

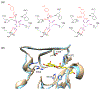
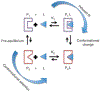
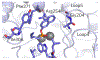

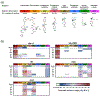
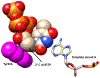
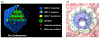
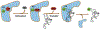
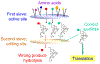
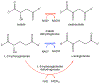
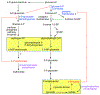
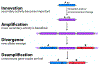
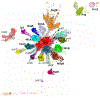
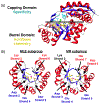
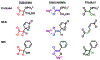

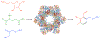
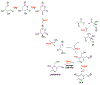
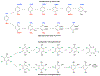
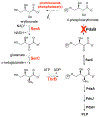
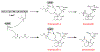
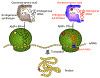
Similar articles
-
An evolutionary biochemist's perspective on promiscuity.Trends Biochem Sci. 2015 Feb;40(2):72-8. doi: 10.1016/j.tibs.2014.12.004. Epub 2015 Jan 5. Trends Biochem Sci. 2015. PMID: 25573004 Free PMC article.
-
Macromolecular crowding: chemistry and physics meet biology (Ascona, Switzerland, 10-14 June 2012).Phys Biol. 2013 Aug;10(4):040301. doi: 10.1088/1478-3975/10/4/040301. Epub 2013 Aug 2. Phys Biol. 2013. PMID: 23912807
-
Protein promiscuity: drug resistance and native functions--HIV-1 case.J Biomol Struct Dyn. 2005 Jun;22(6):615-24. doi: 10.1080/07391102.2005.10531228. J Biomol Struct Dyn. 2005. PMID: 15842167
-
Shining a light on enzyme promiscuity.Curr Opin Struct Biol. 2017 Dec;47:167-175. doi: 10.1016/j.sbi.2017.11.001. Epub 2017 Nov 21. Curr Opin Struct Biol. 2017. PMID: 29169066 Review.
-
Enzyme Promiscuous Activity: How to Define it and its Evolutionary Aspects.Protein Pept Lett. 2020;27(5):400-410. doi: 10.2174/0929866527666191223141205. Protein Pept Lett. 2020. PMID: 31868141 Review.
Cited by
-
Underground metabolism facilitates the evolution of novel pathways for vitamin B6 biosynthesis.Appl Microbiol Biotechnol. 2021 Mar;105(6):2297-2305. doi: 10.1007/s00253-021-11199-w. Epub 2021 Mar 4. Appl Microbiol Biotechnol. 2021. PMID: 33665688 Free PMC article. Review.
-
General Theory of Specific Binding: Insights from a Genetic-Mechano-Chemical Protein Model.Mol Biol Evol. 2022 Nov 3;39(11):msac217. doi: 10.1093/molbev/msac217. Mol Biol Evol. 2022. PMID: 36208205 Free PMC article.
-
Evolution and diversification of carboxylesterase-like [4+2] cyclases in aspidosperma and iboga alkaloid biosynthesis.Proc Natl Acad Sci U S A. 2024 Feb 13;121(7):e2318586121. doi: 10.1073/pnas.2318586121. Epub 2024 Feb 6. Proc Natl Acad Sci U S A. 2024. PMID: 38319969 Free PMC article.
-
A mechanistic view of enzyme evolution.Protein Sci. 2020 Aug;29(8):1724-1747. doi: 10.1002/pro.3901. Protein Sci. 2020. PMID: 32557882 Free PMC article. Review.
-
Paracatalytic induction: Subverting specificity in hedgehog protein autoprocessing with small molecules.Methods Enzymol. 2023;685:1-41. doi: 10.1016/bs.mie.2023.03.001. Epub 2023 Apr 19. Methods Enzymol. 2023. PMID: 37245899 Free PMC article.
References
-
- Martinez-Martinez M, Coscolin C, Santiago G, Chow J, Stogios PJ, Bargiela R, et al. Determinants and prediction of esterase substrate promiscuity patterns. ACS Chem Biol. 2018;13(1):225–34. - PubMed
-
- Zhang X, Ren J, Yao P, Gong R, Wang M, Wu Q, et al. Biochemical characterization and substrate profiling of a reversible 2,3-dihydroxybenzoic acid decarboxylase for biocatalytic Kolbe-Schmitt reaction. Enzyme Microb Technol. 2018;113:37–43. - PubMed
Grants and funding
LinkOut - more resources
Full Text Sources